Abstract
Objective
The purpose of this study was to investigate the isolation and characterization of multipotent human periodontal ligament (PDL) stem cells and to assess their ability to differentiate into bone, cartilage, and adipose tissue.
Methods
PDL stem cells were isolated from 7 extracted human premolar teeth. Human PDL cells were expanded in culture, stained using anti-CD29, -CD34, -CD44, and -STRO-1 antibodies, and sorted by fluorescent activated cell sorting (FACS). Gingival fibroblasts (GFs) served as a positive control. PDL stem cells and GFs were cultured using standard conditions conducive for osteogenic, chondrogenic, or adipogenic differentiation.
Results
An average of 152.8 ± 27.6 colony-forming units was present at day 7 in cultures of PDL stem cells. At day 4, PDL stem cells exhibited a significant increase in proliferation (p < 0.05), reaching nearly double the proliferation rate of GFs. About 5.6 ± 4.5% of cells in human PDL tissues were strongly STRO-1-positive. In osteogenic cultures, calcium nodules were observed by day 21 in PDL stem cells, which showed more intense calcium staining than GF cultures. In adipogenic cultures, both cell populations showed positive Oil Red O staining by day 21. Additionally, in chondrogenic cultures, PDL stem cells expressed collagen type II by day 21.
Many researchers have shown that bone marrow-derived cells can be differentiated into various mesenchymal or connective tissues,1-3 leading to recent investigations focusing on the ability of stem cells to repair damaged tissues. Stem cell studies of the maxillofacial area have evaluated the effects of the regeneration of cartilage and bone tissues in temporomandibular joints,4 the regeneration of periodontal tissues,5-8 and bone regeneration in oral cancer defects using stem cell and gene therapy.9
Stem cells can be classified as mesenchymal cells (MCs; referring to mesenchymal stem cells at the prenatal stage) or mesenchymal stem cells (MSCs; referring to mesenchymal stem cells after birth).10 MCs are known to be the best for the regeneration of all human tissues; however, due to technical difficulties and bioethical arguments, MC research has been met with significant limitations; therefore, adult MSCs are frequently used for research.11 Adult MSCs play a major role in physiological tissue remodeling and are also able to induce the regeneration of damaged tissues. These processes can be applied in various fields. However, since MSCs have to be collected from the bone marrow,12 researchers have developed novel methods (albeit complicated and limited) that utilize MSCs from fascia and fat tissues.13
Homeostasis between teeth and the alveolar bone is maintained through continuous remodeling of the periodontal ligament (PDL), even after orthodontic tooth movement.14,15 PDLs are connective tissues coupling the space between the tooth's cementum and the alveolar bone. They maintain the tooth at a regular position within the alveolar bone, supply nutrition to the surface of roots, and are key factors involved in the regeneration of damaged roots and alveolar bone. Replacement root resorption during childhood results in PDL loss, which can result in defective eruption and ankylosed teeth. These defective teeth may then have a lower occlusal surface than proximal teeth (infraocclusion). PDLs also stabilize the position of teeth and participate in alveolar bone growth and development, remodeling themselves throughout their lifetime. Recent studies have suggested that the PDL space may contain MSCs that are able to differentiate into cementoblasts and osteoblasts, producing mineral tissues and fibroblasts that form fibrous connective tissues.16 These MSCs are thought to originate from capillaries in bone tissues and the PDL space, ultimately differentiating in the PDLspace.16 Therefore, teeth and PDLs have emerged as potential important sources of undifferentiated MSCs, even in adults, where acquisition of MSCs is limited to the bone marrow and fascia.
In the dental field, Gronthos et al.17 reported the successful isolation and differentiation of undifferentiated MSCs from surgically extracted mandibular third molars. Since then, many researchers have also successfully isolated MSCs from uninfected impacted third molars and dental pulp tissues.18-23 However, all of these studies are restricted to fairly young, unerupted third molars, which are not easy to obtain from general dental clinics.
The aim of this research was to determine whether undifferentiated MSCs isolated from the root surface of adult premolars extracted for orthodontic treatment could be cultured to differentiate into bone, cartilage, and fat tissue.
Maxillary first premolars were extracted from 7 patients (5 men and 2 women, ages 20.3 - 28.4 years) before orthodontic treatment. All procedures were approved by the ethics committee of Pusan National University Hospital Medical Institute (2008-2) and were performed after obtaining informed consent agreements from patients. Tissues from PDLs were carefully excised with a scalpel from the middle third of freshly extracted teeth. The PDL tissue was digested in 3 mg/mL collagenase type I (Invitrogen, Carlsbad, CA, USA) and 4 mg/mL dispase (Invitrogen) for 1 h at 37℃. Single-cell suspensions were obtained with a 70-µm cell strainer (Falcon BD, Franklin Lakes, NJ, USA). To separate cells expected to be stem cells, we allowed suspensions of 1 × 104 cells to attach to 100-mm dishes (NUNC, Roskilde, Denmark) in alpha modification of Eagle's medium (alpha-MEM; Gibco BRL, Carlsbad, CA, USA) supplemented with 10% fetal bovine serum (FBS; Gibco BRL), 100 mM ascorbic acid 2-phosphate(Sigma, St. Louis, MO, USA), 2 mM glutamine, 100 U/mL penicillin, and 100 mg/mL streptomycin (Gibco BRL). Cells were then cultured for 10 days in 5% carbon dioxide at 37℃. Gingival fibroblasts (GFs) derived from the gingival tissue attached to the cementoenamel junctions of the extracted premolars were used as controls for cell proliferation assays. All cells used for further assays were second or third passage cells.
For colony formation assays, 1 × 104 cells were plated in a 100-mm petridish and cultured for 7 days. After fixation for 20 min with 5 mL of 4% paraformaldehyde, cells were stained with 0.05% crystal violet to assess colony-formation efficiency. Fifty or more cells clustered together were considered a colony.
Cell suspensions from single cells were acquired using a 70-µm cell strainer (Falcon BD). Next, 1 × 105 cells were added to a FACS tube in blocking buffer (HBSS, 20 mM HEPES, 1% normal human serum, 1% bovine serum albumin, 5% FBS), and 10 µL of anti-STRO-1 immunoglobulin (Ig)M (Santa Cruz Biotechnology, Santa Cruz, CA, USA). Anti-human CD29 (integrin β-1 receptor marker for cell proliferation and adhesion; BD Bioscience, Sparks, MD, USA), anti-human CD34 (specific hematopoietic stem cell marker; BD Bioscience), and anti-human CD44 (hyaluronate receptor that facilitates adhesion to stromal progenitors during cell culture but not to hematopoietic progenitors; BD Bioscience) antibodies were also added. Cells were then cultured for 30 min at 4℃. Anti-mouse K antibodies were used as a negative control. After primary antibody incubation, goat anti-mouse IgM FITC secondary antibodies (2 µg/mL; Molecular Probes, Eugene, Carlsbad, CA, USA) were added, and cells were incubated for an additional 1 h. Positively stained cells were separated by flow cytometry and analyzed with the automated cell deposition unit FACScan (Becton Dickinson, Parsippany, NJ, USA) at 4℃. For comparison of MSC marker expression, all experiments were performed separately for each patient.
To estimate cell proliferation capacity, 1 × 104 cells were seeded and cultured in DMEM media containing 20% FBS and 1% antibiotics for 1 week. Coulter cell counters (Coulter Electronics Inc., Hialeah, FL, USA) were used to determine the number of cells.
Seven dishes of 3 × 104 cells each were seeded and cultured for 3 days. Osteogenesis-inducing media (α-MEM containing 1% acetic acid, 10% FBS, 0.1 µM dexamethasone, 50 µM ascorbic acid 2-phosphate, and 10 mM β-glycerophosphate) was changed every 2 - 3 days for 3 weeks to induce osteogenesis. After 3 weeks, the culture was washed 3 times with phosphate buffered saline (PBS) and fixed for 15 min with 4% paraformaldehyde. One hundred microliters of substrate solution was added, and cells were cultured for 30 min at 37℃. Next, 100 µL stop solution (0.9 N NaOH) was added, and calcium formation was determined by 2% Alizarin red S staining (300 µL; Sigma-Aldrich) after a 15-min fixation with 70% ethanol and washing with PBS.
Cells were seeded into 7 dishes and cultured for 3 days. Adipogenesis-inducing media (α-MEM containing 1% acetic acid, 10% FBS, 0.5 mM isobutylmethylxanthine, 1 µM dexamethasone, 10 µM insulin, and 100 µM indomethacin) was changed every 2 - 3 days for 3 weeks to induce lipogenesis. After 3 weeks, cultures were washed 3 times with PBS, fixed for 15 min with 4% paraformaldehyde, and then washed 3 more times with PBS. The dishes were stained for 2 h at room temperature with 3% Oil Red O (Sigma-Aldrich). After washing the cultures 3 times with PBS, photographs were taken. Staining was quantified by the addition of 500 µL isopropanol after drying.
To induce chondrogenesis, 3 × 105 cultured cells were placed into a 15-mL tube for centrifugation. Chondrogenic inducing media (Cambrex Bio Science, Charles City, IA, USA) with 10 ng/mL transforming growth factor (TGF)-β3 (Cambrex Bio Science) was changed every 2 - 3 days for 3 weeks. Collagen type II immunohistochemistry was performed to check collagen formation. Culture tubes were frozen for 30 min and fixed with acetone for 10 min. After drying, the culture was washed twice with PBS and then primary antibodies (collagen type II, Sigma-Aldrich) were added. After 24 h, secondary antibodies were used.
Differences in the number of colony forming units (CFUs) between PDL stem cells and GFs were compared using t-tests. Sexual differences between donors were not shown to be significant due to the small sample sizes. Statistics were calculated using SPSS for Windows (version 16.0; SPSS Inc., Chicago, IL, USA).
Fifty or more cells clustered together were considered a CFU. Control GFs exhibited an average of 26.3 ± 13.4 CFUs after 7 days, while undifferentiated MSCs exhibited an average of 152.8 ± 27.6 CFUs (Figures 1 and 2). As shown in Figure 1, there was a statistically significant difference in the total number of CFUs between PDL (n = 7) and GF (n = 7) cultures. In addition, we analyzed the cell proliferation rate over the course of 6 days and found that the proliferation rate of PDL stem cells was over 2 times higher than GFs at day 4 and nearly 3 times higher at day 6 (Figure 3).
FACS analysis was performed using antibodies specific for undifferentiated MSCs. CD29 and CD44 were expressed in an average of 52.2 ± 21.4% and 99.6 ± 0.2% of cells, respectively. In contrast, CD34-positive cells were found at a very low rate of 0.5 ± 0.4%. An average of 5.6 ± 4.5% of cells were positive for the stem cell-specific marker, STRO-1 (Table 1 and Figure 4).
After culturing PDL cells and GFs in osteogenesis-inducing media for 21 days, calcified crystals were observed in both cell lines. PDL cells in particular contained an abundance of calcified crystals. In contrast, both cell types showed no formation of calcified crystals when cultured with normal media (Figure 5).
Chondrogenesis was induced by culturing cells in specific differentiating media for 21 days. Immunohistochemical staining for collagen type II revealed positive staining in all cell groups. Furthermore, when cultured in chondrogenesis-inducing media, both PDL cells and GFs demonstrated chondrogenic induction with metachromasia and cell morphology corresponding to embryonic stages of cartilage formation (Figure 6).
Following culture in adipogenesis-inducing media for 21 days, both PDL cells and GFs were positively stained for an adipose tissue marker. Staining was faint in GFs, and both cell types exhibited no evidence of adipogenic induction after 21 days of culture in normal media (Figure 7).
Many researchers have investigated the potential applications of tissue engineering for restoration of teeth and periodontal tissues after suffering disease or trauma. Indeed, there has been a huge focus on studies examining potential methods for grafting and successful biological substitutes. However, these methods have failed to result in successful tissue regeneration.16 To overcome this limitation, tissue regeneration using stem cells is now being studied. However, stem cell-related work is limited by ethical issues surrounding embryonic stem cell research as well as experimental challenges related to the limited supply of adult stem cells. Therefore, the discovery of new, robust sources of stem cells is a major focus of many researchers.11-13 While adult stem cells do not exhibit totipotency like embryonic stem cells, they still have regenerative, proliferative, and differentiating potential. Adult stem cells can be divided into hematopoietic cells and undifferentiated MSCs according to their differentiating potential. Undifferentiated MSCs have the ability to differentiate into specific cells and possess clonogenic potential, that is, the potential for a single cell to form a cluster of identical cells.12 Undifferentiated MSCs become progenitor cells, i.e., partially differentiated cells (in morphogenic terms), before finally progressing into mature cells.24-27 The PDL space contains massive amounts of fibroblasts, osteoblasts, and cementoblasts for regeneration of PDL tissues. Recent studies have shown that PDL spaces contain progenitor cells, which are assumed to differentiate into these various cells. However, it is still unclear whether these cells are progenitors or undifferentiated MSCs that have the potential to become any kind of cell. The purpose of this study was to determine whether undifferentiated MSCs exist on the adult premolar root surface and, furthermore, to determine whether these cells could differentiate into various progenitor cells in vitro.
In this study, we collected PDL cells from the maxillary first premolar root surface and cultured these cells for 2 passages. Seven days later, CFUs were counted following culture in stem cell-specific medium. Culturing cells at a low density (1 × 104) for 7 days resulted in the growth of over 150 CFUs. In a previous study, Seo et al.18 also reported the isolation of over 170 single colonies assumed to be stem cells after isolation of PDLs from the third molar. Single cells exhibited morphologies similar to fibroblasts when visualized by light microscopy. Moreover, Pittenger et al.3 demonstrated that undifferentiated MSCs are similar to fibroblasts in terms of morphology, but exhibit differences in their replicating or proliferative potential. In this study, the proliferative potential of cultured PDL cells from day 3 showed a nearly 2.5-fold increase in proliferation when compared to fibroblasts on day 6, indicating that the isolated PDL cells contained undifferentiated MSCs.
The most common way to collect undifferentiated MSCs is from the bone marrow.12 However, cells from the bone marrow are mostly hematopoietic stem cells, and only approximately 0.1% of these cells are undifferentiated MSCs. Therefore, separating these 2 cell populations is a major challenge.3 A common method to separate undifferentiated MSCs is flow cytometry using FACS. FACS uses undifferentiated MSC-specific surface antibodies to mark cells with fluorescence or differential light absorbance for isolation ("sorting").28 Therefore, it is important to identify and utilize appropriate markers for undifferentiated MSCs during FACS analysis. A commonly used undifferentiated MSC-specific marker is STRO-1. Mouse-raised IgM STRO-1 antibodies do not respond to hematopoietic stem cells, but instead attach to a surface antigen expressed on progenitor MSCs in human bone marrow. According to experiments performed by Simmons and Torok-Storb,29 STRO-1-positive cells are morphologically similar to fibroblast clonogenic CFUs. However, erythrocyte progenitor cells can also stain positive for STRO-1; therefore, most tests are conducted using several surface antibodies simultaneously. In this study, we also used anti-CD29 (integrin β-1 receptor),30 -CD44 (hyaluronate receptor),30 and -CD34 (hematopoietic stem cell marker)31,32 antibodies simultaneously. Baddoo et al.30 reported that undifferentiated MSCs from bone marrow express both CD29 and CD44. Therefore, MSCs can be collected by screening out CD29- and CD44-negative cells. Additional reports have shown that CD34 is expressed on hematopoietic stem cells during culture, but not on MSCs, making it a useful screening tool. Using flow cytometry analysis, we found that between 2.6% and 14.7% of second-passage PDL-derived cells were positive for STRO-1. Moreover, these cells exhibited an average of 52.2%, 99.6%, and 0.5% cells that were positive for CD29, CD44, and CD34, respectively. In this analysis, however, staining with CD29 antibodies resulted in a larger standard deviation (i.e., 52.2 ± 21.4%) than staining with other antibodies. Thus, it is possible that CD29 is expressed in all proliferating cells within PDL cell pools. Gay et al.19 reported that an average of 27% of PDL-derived cells were STRO-1-positive, this was higher than the percentage of positively stained cells in our study. These differences may be related to the sources of MSCs as Gay's study used the PDL and dental follicles of an impacted third molar with an immature root end. In addition, Sawa et al.33 insisted that differences in the PDL cell cycle of an immature permanent tooth and a fully matured one were the result of aging. This supports our data because all of our sources of PDL cells were from fully matured maxillary premolars from adults older than 20 years. Unfortunately, the correlation between age and the number of undifferentiated MSCs was not examined in this study. Further studies with a larger data pool would be required to investigate this.
To determine if PDL cells differentiate successfully, calcium production was tested after culturing with osteogenesis inducing media for three weeks. This study resulted in overall high production of calcium in PDL cells from osteogenesis inducing media and from some GF cells as well. However, cultured in normal media, PDL cells and GF cells did not produce calcium. This result coincides with Gronthos et al.,17 Seo et al.,18 Gay et al.,19 Shi et al.20 Many various factors are required for an undifferentiated MSC to differentiate into osteoblast or cementoblast cells. Studies show that dexamethasone and ascorbic acid have a close relationship with the differentiation of osteoblasts. Our osteogenesis inducing media contained these substances as well. However, experiments carried out in vivo used bone morphogenic protein 2 (BMP2) more frequently.34,35 For clinical use, studies of growth factors like BMP2 are required.
To determine whether PDL cells have the potential to differentiate into various mature tissues, we first investigated whether they could form adipose tissue using Oil red O staining of PDL cells grown for 3 weeks in lipogenesis-inducing growth media. The main lipogenesis-inducing component was indomethacin, which acts as an antagonist to the peroxisome proliferator-activator receptor (PPAR) gamma on the surface of undifferentiated MSCs, thereby activating lipoprotein lipase within adipose-forming cells.3 Lecka-Czernik36 also demonstrated that application of PPAR gamma 2 to odontoblasts allowed these cells to differentiate into adipose tissue. However, in this study, we were unable to isolate and identify adipose tissues. Thus, further studies are required in order to shed more light on this process.
Cartilage tissues generally do not exist in GF cells. However, it is important to confirm whether cartilage tissues could form from undifferentiated MSCs of PDL origin since MSC-derived cartilage tissues could be used in temporomandibular joint disc regeneration. By using collagen type II staining, we demonstrated that both GFs and PDL cells could form cartilage tissues when cultured in TGFβ3-containing, cartilage-inducing media. TGFβ3 is known to be a major factor involved in cartilage formation in MSCs. Preliminary studies have demonstrated that these cells do not exhibit cartilage formation in normal media. However, through aggregation of cells by centrifugation and culturing with appropriate inducing media and TGFβ3, cartilage formation was induced. We expect that this is a result of the interaction between proteoglycans secreted from cells of connective tissues. Further studies are required to develop and adapt techniques for tissue engineering of cartilage cells.
In this study, we sought to identify whether PDL cells could be classified as undifferentiated MSCs and to test their potential to differentiate into bone, cartilage, and fat tissues. From this study, we found that PDL cells, collected and isolated from the maxillary first premolars of 7 patients, exhibited a 2-fold greater proliferation rate than GFs. We also successfully identified a certain amount of undifferentiated MSCs in the pool of PDL cells and confirmed that these cell populations could form bone, adipose, and cartilage tissues. These findings suggested that periodontal ligament cells from extracted adult premolars may be useful sources of stem cells for oral and maxillofacial tissue engineering in the future.
Figures and Tables
Figure 1
Cell culture colony-forming units (CFUs). At day 7 after plating, PDL cells were fixed with 10% formalin and stained with 4% crystal violet. Aggregates of 50 or more cells were considered colonies and counted. A, A representative GF colony (10×). B, A representative PDL cell colony (10×). The black arrows and circles represent the images of 7-day cell population (right panels) at 10× magnification using light microscopy.
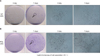
Figure 2
Colony forming units (CFUs) in gingival fibroblasts (GFs) (n = 7) and periodontal ligament (PDL) cells (n = 7). Colony formation was measured as described in the Material and Methods section. PDL cells exhibited a statistically significant increase in CFUs as compared to GFs, the positive control. *p < 0.05.
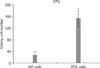
Figure 3
Cell proliferation rates. Periodontal ligament (PDL) cells and gingival fibroblasts (GFs) were cultured for 1, 2, 3, 4, 5, and 6 days in 6-well plates with media changes every other day. The cells were harvested by trypsinization and counted with a Coulter counter. The data are from 1 of 7 representative experiments, each yielding similar results. From day 4, PDL cell proliferation was significantly increased as compared to GFs, the positive control. Diamonds correspond to PDL cells, and squares correspond to GFs. *p < 0.05, †p < 0.01.
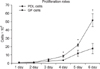
Figure 4
Primary digested pooled adult human periodontal ligament cells were immunostained with various antibodies, and cells were analyzed on a FACScan with an automated cell deposition unit equipped with an argon laser. The data were analyzed with Cell Quest software. These representative FACScan cell distributions were collected from a passage 2 cell population from Donor 1. Percentages of CD29-positive (A), CD34-positive (B), CD44-positive (C), and STRO-1-positive (D) cells were identified and are indicated by the circle in each corresponding graph.
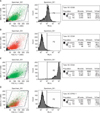
Figure 5
Expression of mineral deposits within periodontal ligament (PDL) cells and gingival fibroblasts (GFs) after long-term culture in osteogenesis-inducing media. A, C, No calcium deposits were seen in PDL cells and GFs cultured in normal media (20×). B, GFs cultured with osteogenesis-inducing media exhibited relatively small calcium deposits at day 21; the distribution was observed as weak foci associated with cell clusters (20×). D, At day 21, PDL cells cultured with osteogenesis-inducing media showed mineral deposits as evidenced by Alizarin red S staining (20×), with deposits distributed throughout the tissue culture well.
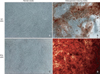
Figure 6
In vitro chrondogenic differentiation of periodontal ligament (PDL) cells and gingival fibroblasts (GFs) as measured by immunohistochemical staining for collagen type II expression. Panels A and B show GFs cultured in normal DMEM or chondrogenic differentiation medium, respectively, after 3 weeks (10×). Panels C and D show PDL cell cultures after 3 weeks of incubation in α-MEM containing ascorbic acid and glutamine and chondrogenic differentiation medium, respectively (10×). In the cell populations shown in panels B and D above, metachromasia and cell morphology corresponding with embryonic stages of cartilage formation were observed.
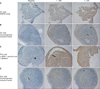
Figure 7
Characterization of the adipogenic differentiation of periodontal ligament (PDL) stem cell and gingival fibroblast (GF) cultures (20×). A, Lipid droplets were not seen in PDL cells cultured with normal media. B, Weak lipid droplets were seen in GFs cultured with adipogenesis-inducing media (100×). C, Lipid droplets were not observed in PDL cells cultured for 21 days in normal media, as is shown by negative oil red O staining (20×). D, PDL cells formed lipid droplets after 21 days of culture in adipogenesis-inducing media (100×).

Notes
References
1. Friedenstein AJ, Chailakhjan RK, Lalykina KS. The development of fibroblast colonies in monolayer cultures of guinea-pig bone marrow and spleen cells. Cell Tissue Kinet. 1970. 3:393–403.


3. Pittenger MF, Mackay AM, Beck SC, Jaiswal RK, Douglas R, Mosca JD, et al. Multilineage potential of adult human mesenchymal stem cells. Science. 1999. 284:143–147.


4. Alhadlaq A, Mao JJ. Tissue-engineered osteochondral constructs in the shape of an articular condyle. J Bone Joint Surg Am. 2005. 87:936–944.


5. Doğan A, Ozdemir A, Kubar A, Oygür T. Healing of artificial fenestration defects by seeding of fibroblast-like cells derived from regenerated periodontal ligament in a dog: a preliminary study. Tissue Eng. 2003. 9:1189–1196.


6. Nakahara T, Nakamura T, Kobayashi E, Kuremoto K, Matsuno T, Tabata Y, et al. In situ tissue engineering of periodontal tissues by seeding with periodontal ligament-derived cells. Tissue Eng. 2004. 10:537–544.


7. Akizuki T, Oda S, Komaki M, Tsuchioka H, Kawakatsu N, Kikuchi A, et al. Application of periodontal ligament cell sheet for periodontal regeneration: a pilot study in beagle dogs. J Periodontal Res. 2005. 40:245–251.


8. Hasegawa M, Yamato M, Kikuchi A, Okano T, Ishikawa I. Human periodontal ligament cell sheets can regenerate periodontal ligament tissue in an athymic rat model. Tissue Eng. 2005. 11:469–478.


9. Nussenbaum B, Rutherford RB, Teknos TN, Dornfeld KJ, Krebsbach PH. Ex vivo gene therapy for skeletal regeneration in cranial defects compromised by postoperative radiotherapy. Hum Gene Ther. 2003. 14:1107–1115.


10. Sloan AJ, Smith AJ. Stem cells and the dental pulp: potential roles in dentine regeneration and repair. Oral Dis. 2007. 13:151–157.


11. Young HE, Duplaa C, Katz R, Thompson T, Hawkins KC, Boev AN, et al. Adult-derived stem cells and their potential for use in tissue repair and molecular medicine. J Cell Mol Med. 2005. 9:753–769.


12. Kemp KC, Hows J, Donaldson C. Bone marrow-derived mesenchymal stem cells. Leuk Lymphoma. 2005. 46:1531–1544.


13. Sakaguchi Y, Sekiya I, Yagishita K, Muneta T. Comparison of human stem cells derived from various mesenchymal tissues: superiority of synovium as a cell source. Arthritis Rheum. 2005. 52:2521–2529.


14. Müssig E, Tomakidi P, Steinberg T. Molecules contributing to the maintenance of periodontal tissues. Their possible association with orthodontic tooth movement. J Orofac Orthop. 2005. 66:422–433.


15. Krishnan V, Davidovitch Z. Cellular, molecular, and tissue-level reactions to orthodontic force. Am J Orthod Dentofacial Orthop. 2006. 129:469.e1–469.e32.


16. Mao JJ, Giannobile WV, Helms JA, Hollister SJ, Krebsbach PH, Longaker MT, et al. Craniofacial tissue engineering by stem cells. J Dent Res. 2006. 85:966–979.


17. Gronthos S, Mankani M, Brahim J, Robey PG, Shi S. Postnatal human dental pulp stem cells (DPSCs) in vitro and in vivo. Proc Natl Acad Sci U S A. 2000. 97:13625–13630.
18. Seo BM, Miura M, Gronthos S, Bartold PM, Batouli S, Brahim J, et al. Investigation of multipotent postnatal stem cells from human periodontal ligament. Lancet. 2004. 364:149–155.


19. Gay IC, Chen S, MacDougall M. Isolation and characterization of multipotent human periodontal ligament stem cells. Orthod Craniofac Res. 2007. 10:149–160.


20. Shi S, Bartold PM, Miura M, Seo BM, Robey PG, Gronthos S. The efficacy of mesenchymal stem cells to regenerate and repair dental structures. Orthod Craniofac Res. 2005. 8:191–199.


21. Jo YY, Lee HJ, Kook SY, Choung HW, Park JY, Chung JH, et al. Isolation and characterization of postnatal stem cells from human dental tissues. Tissue Eng. 2007. 13:767–773.


22. Laino G, d'Aquino R, Graziano A, Lanza V, Carinci F, Naro F, et al. A new population of human adult dental pulp stem cells: a useful source of living autologous fibrous bone tissue (LAB). J Bone Miner Res. 2005. 20:1394–1402.


23. Miura M, Gronthos S, Zhao M, Lu B, Fisher LW, Robey PG, et al. SHED: stem cells from human exfoliated deciduous teeth. Proc Natl Acad Sci U S A. 2003. 100:5807–5812.


24. Digirolamo CM, Stokes D, Colter D, Phinney DG, Class R, Prockop DJ. Propagation and senescence of human marrow stromal cells in culture: a simple colony-forming assay identifies samples with the greatest potential to propagate and differentiate. Br J Haematol. 1999. 107:275–281.


25. Reyes M, Lund T, Lenvik T, Aguiar D, Koodie L, Verfaillie CM. Purification and ex vivo expansion of postnatal human marrow mesodermal progenitor cells. Blood. 2001. 98:2615–2625.


26. Galotto M, Campanile G, Robino G, Cancedda FD, Bianco P, Cancedda R. Hypertrophic chondrocytes undergo further differentiation to osteoblast-like cells and participate in the initial bone formation in developing chick embryo. J Bone Miner Res. 1994. 9:1239–1249.


27. Bennett JH, Joyner CJ, Triffitt JT, Owen ME. Adipocytic cells cultured from marrow have osteogenic potential. J Cell Sci. 1991. 99:131–139.


28. Majumdar MK, Thiede MA, Mosca JD, Moorman M, Gerson SL. Phenotypic and functional comparison of cultures of marrow-derived mesenchymal stem cells (MSCs) and stromal cells. J Cell Physiol. 1998. 176:57–66.


29. Simmons PJ, Torok-Storb B. Identification of stromal cell precursors in human bone marrow by a novel monoclonal antibody, STRO-1. Blood. 1991. 78:55–62.


30. Baddoo M, Hill K, Wilkinson R, Gaupp D, Hughes C, Kopen GC, et al. Characterization of mesenchymal stem cells isolated from murine bone marrow by negative selection. J Cell Biochem. 2003. 89:1235–1249.


31. Lemoli RM, Tafuri A, Fortuna A, Catani L, Rondelli D, Ratta M, et al. Biological characterization of CD34+ cells mobilized into peripheral blood. Bone Marrow Transplant. 1998. 22:Suppl 5. S47–S50.
32. Waller EK, Olweus J, Lund-Johansen F, Huang S, Nguyen M, Guo GR, et al. The "common stem cell" hypothesis reevaluated: human fetal bone marrow contains separate populations of hematopoietic and stromal progenitors. Blood. 1995. 85:2422–2435.


33. Sawa Y, Phillips A, Hollard J, Yoshida S, Braithwaite MW. The in vitro life-span of human periodontal ligament fibroblasts. Tissue Cell. 2000. 32:163–170.


34. Hou LT, Li TI, Liu CM, Liu BY, Liu CL, Mi HW. Modulation of osteogenic potential by recombinant human bone morphogenic protein-2 in human periodontal ligament cells: effect of serum, culture medium, and osteoinductive medium. J Periodontal Res. 2007. 42:244–252.

