Abstract
Certain limitations of the neurosphere assay (NSA) have resulted in a search for alternative culture techniques for brain tumor-initiating cells (TICs). Recently, reports have described growing glioblastoma (GBM) TICs as a monolayer using laminin. We performed a side-by-side analysis of the NSA and laminin (adherent) culture conditions to compare the growth and expansion of GBM TICs. GBM cells were grown using the NSA and adherent culture conditions. Comparisons were made using growth in culture, apoptosis assays, protein expression, limiting dilution clonal frequency assay, genetic affymetrix analysis, and tumorigenicity in vivo. In vitro expansion curves for the NSA and adherent culture conditions were virtually identical (P=0.24) and the clonogenic frequencies (5.2% for NSA vs. 5.0% for laminin, P=0.9) were similar as well. Likewise, markers of differentiation (glial fibrillary acidic protein and beta tubulin III) and proliferation (Ki67 and MCM2) revealed no statistical difference between the sphere and attachment methods. Several different methods were used to determine the numbers of dead or dying cells (trypan blue, DiIC, caspase-3, and annexin V) with none of the assays noting a meaningful variance between the two methods. In addition, genetic expression analysis with microarrays revealed no significant differences between the two groups. Finally, glioma cells derived from both methods of expansion formed large invasive tumors exhibiting GBM features when implanted in immune-compromised animals. A detailed functional, protein and genetic characterization of human GBM cells cultured in serum-free defined conditions demonstrated no statistically meaningful differences when grown using sphere (NSA) or adherent conditions. Hence, both methods are functionally equivalent and remain suitable options for expanding primary high-grade gliomas in tissue culture.
The demonstration of adult mammalian neural stem cells (NSCs) in the 1990s [1, 2, 3] confirmed the suspicions of previous investigators, that sustained cell genesis in the adult central nervous system (CNS) was due to the existence of a CNS stem cell [4, 5, 6, 7, 8, 9, 10, 11, 12]. The original isolation and expansion of NSCs used a tissue culture method that deviated from other protocols. First, the growth medium was defined (i.e., serum-free) and second no substrate (i.e., poly-L-ornithine or laminin) was provided. Instead, cells were cultured on tissue culture plastic. The addition of epidermal growth factor (EGF) stimulated the proliferation of precursor cells (NSCs and progenitor cells) resulting in the formation of clonal spheres, referred to as neurospheres. The neurospheres could be serially passage resulting in an arithmetic increase in the total number of cells generated over time. This culture methodology is referred to as the neurosphere assay and is a well accepted in vitro approach to isolating and expanding NSCs.
While useful for the maintenance and study of somatic stem cells, the neurosphere assay has subsequently been used to isolate and expand tumor cells [13, 14, 15, 16, 17]. Based on the pioneering work of the Dick lab and their characterization of a stem-like cell driving proliferation of acute myeloid leukemia [18], the origin, maintenance, and resistance of solid tissue malignancies, including malignant gliomas, are attributed to transformed precursors that have the cardinal properties of stem cells [19, 20]. A body of evidence now exists suggesting that brain tumors contain this sub-population of tumor-initiating cells (TICs) that exhibit stem cell characteristics [13, 14, 20, 21]. Moreover, this literature suggests that this population may be responsible for treatment resistance [22, 23, 24], and targeting this population may be an important therapeutic strategy in treating patients with brain tumors [20, 25]. Therefore, studying malignant glioma in culture requires the culture conditions to maintain the TICs that are hypothesized to drive tumor growth, as well as preserve the genetic and phenotypic properties of these cells. Only with these criteria can cell culture results be relevant for patients with glioblastoma multiforme (GBM).
Given the potential similarity between somatic NSCs and cancer-like stem cells or TICs, Ignatova et al. [21] were the first to use the neurosphere assay (NSA) to isolate and expand cells from adult human brain tumors. This was quickly followed by detailed reports further characterizing the stem cell properties of a sub-population of cells within human brain tumors and their ability to initiate tumor formation in vivo [13, 14] and has rapidly become the standard for identifying and maintaining brain TICs in culture [26]. One of the reasons for the broad acceptance of this methodology is the retention of the primary tumor's phenotype following culture and xenografting into a murine model [13, 27]. In addition, brain TICs have been found to be more representative of the original tumor genetically when grown in the NSA compared to serum conditions even after serial passage in vitro [27, 28].
However, this assay has notable limitations such as variable composition of cells and overestimation of the proportion of NSCs. First, the NSA can produce variable composition of cell types depending on the media [29], frequency of passaging and whether dissociation is performed before cell differentiation [30]. Moreover, the assay can overestimate the number of stem cells [31]. A 1:1 relationship between stem cell and neurosphere does not exist and the NSA overestimates the proportion of NSCs (usually <5% of the overall NSA population) by an order of magnitude [31]. Additionally, neurospheres are not always clonal since they are mobile and can merge with one another [32].
To overcome these limitations, modifications to the NSA have been proposed. The colony-forming assay is a semisolid culture with collagen that has been described to prevent the migration and fusion of mouse NSC spheres [33]. Only cells from the large colonies (>2 mm) demonstrated stem cell characteristics and the capability for long-term self-renewal (>7 passages) [33]. Similarly with human brain TICs, the NSA has been supplemented with methylcellulose in an attempt to decrease sphere motility [21]. Recently, serum free culture conditions supplemented with laminin have been described to grow NSC as a monolayer [34, 35, 36, 37]. These techniques were extended to brain TICs with an extracellular matrix [38] and subsequently laminin [39]. Some of these data suggest that establishing cell lines from human gliomas is more efficient when the cells are grown as a monolayer and that the NSA is inferior to adherent culture methods in terms of higher percentages of apoptosis and differentiation potential of cells [39]. To further investigate this hypothesis we quantitatively compared GBM cells grown in NSA to cells grown in adherent/laminin conditions with respect to their respective phenotypic, genetic and functional characteristics.
With Institutional Review Board (IRB) at the University of Florida approval and written patient consent, fresh brain tumor samples were obtained at the time of surgical excision from adult patients after obtaining informed consent. GBM tumor samples were dissociated into single cells using trypsin and cultured in the NSA at a cell density of 100,000 to 200,000 cells/ml. These samples were established as cell lines by undergoing long-term propagation (>10 passages) in NSA growth media and were subsequently used for NSA versus adherent culture comparisons.
The NSA growth media included human NSC basal medium containing human NeuroCult proliferation supplement (Stem Cell Technologies, Vancouver, BC, Canada) with 20 ng/ml EGF (R&D Systems, Minneapolis, MN, USA), 10 ng/ml basic fibroblast growth factor (R&D Systems) and 2 µg/ml heparin (Sigma, St. Louis, MO, USA). Cultures were grown in T25 flasks. The adherent culture required coating of flasks with laminin (diluted 1:10 in NSC medium, Invitrogen, Carlsbad, CA, USA) for at least 3 hours at 37℃ with 5% CO2. The same medium was used for the adherent and NSA culture conditions. The NSA cells were grown until formation of 100-µm spheres to avoid problems with cell differentiation, death and cellular attachment associated with larger spheres. The adherent cells were grown until cells were confluent per previously described protocol [39]. For passage of cells, the cells were dissociated with 0.05% trypsin-EDTA (Gibco, Grand Island, NY, USA) for 2 minutes at 37℃, counted and re-plated at a density of 50,000 cells/ml. Cells were stained with 0.04% trypan blue at the time of counting to identify dead cells (trypan blue +). These cells were counted and the percentage of dead cells was calculated for each growth condition at the time of each passage. These values were compared using the Student's t test and a P-value of ≤0.05 was considered significant.
Additionally, the fold expansion at each passage was compared between NSA and the laminin growth conditions. The difference was compared to zero using the Student's t test and P-values were calculated. A P-value of ≤0.05 was considered significant.
For apoptosis assays, live cells were evaluated for caspase 3 (1:300, Calbiochem, San Diego, CA, USA), annexin V (1:100, Invitrogen), 1,1',3,3,3',3'-hexamethylindodicarbo-cyanine iodide (DiIC; 1:1,000, Invitrogen), propidium iodide (PI; 1:1,000, Sigma), and 4',6-diamidino-2-phenylindole (DAPI; 1:2,000, Invitrogen). For fixed cells, analysis with caspase 3 (1:1,000, BD, San Diego, CA, USA) was performed with cells fixed with 90% methanol at 20℃ for 15 minutes. For comparison of neurosphere and adherent culture systems, three primary GBM cultures (GB:A1, GB:A2, GB:A3) were evaluated in triplicate for expression of EphA3 (in-house mAb 5 µg/ml, IIIA4), epidermal growth factor receptor (EGFR; in-house mAb 5 µg/ml, 528), EGFRvIII (in-house mAb 5 µg/ml, 806), platelet-derived growth factor receptor α (1:50, R&D Systems), c-Met (LMH-85, in-house mAb 5 µg/ml), HER2 (1:100, Herceptin), CD15 (1:100, BD), CD44 (5 µg/ml, Hermes 3), CD133 (1:10, Miltenyi Biotech, Auburn, CA, USA), CD49f (1:50, Millipore, Billerica, MA, USA), Mcl-1 (1:100, Santa Cruz Biotechnology, Santa Cruz, CA, USA) and IL13Ra2 (in-house mAb 5 µg/ml, Ab12). IgG1 (1:400, BD) was used an isotype control.
The GBM cell lines were maintained in NSA and adherent culture conditions. The cells were fixed and stained with antibodies against differentiation and proliferative markers. Flow cytometry was performed and percent cell staining and geometric mean were measured. Nestin (1:1,000, Chemicon, Temecula, CA, USA), glial fibrillary acidic protein (GFAP; 1:2,000, BD), beta tubulin III (1:2,000, Promega, Madison, WI, USA), MCM2 (1:500, Santa Cruz Biotechnology), and Ki67 (1:750, Invitrogen) were used for analysis of differentiation. Flow cytometry was performed using the LSR II (BD). Flow cytometry analysis was performed with Flow Jo (Treestar Inc., San Carlos, CA, USA). The percent cells positive and geometric mean values are reported as mean±standard error. The values were compared using the Student's t test and computing P-values.
Cells were grown in 96-well plates coated with laminin with dilutions of 1-10 cells/well. The wells that became confluent or close to confluent were considered to have clonogenic cells. For the NSA, cells were plated and wells were evaluated for number of neurospheres at least 100 µm in size (sphere forming frequency). The confluency and size requirements were to ensure determination of true stem-like cells as opposed to progenitor cells with limited proliferative capability [33].
The percent of empty wells (with less than 75% growth for laminin and number of wells with spheres less than the total number of cells plated) were enumerated and the negative log (-log) of the proportion of negative wells was calculated for each cell density. This result was plotted to determine the clonal frequency of the adherent culture conditions using the extreme limiting dilution analysis software [40]. The clonal frequency of the NSA was compared to the adherent cultures using a Mann-Whitney test. These data are reported as a mean with standard error.
GBM cells were grown in the NSA (n=6) or adherent (n=6, representing two cell lines and technical replicates of the experiments) culture conditions, dissociated into single cell suspension and then snap frozen using liquid nitrogen. Total RNA was isolated using RNeasy kit (Qiagen, Valencia, CA, USA) according to manufacturer's protocol with an oncolumn DNase digestion. RNA quality and quantity were assessed using 2100 Bioanalyzer (Agilent Technologies, Santa Clara, CA, USA) and NanoDrop (Thermo Scientific, Waltham, MA, USA), respectively. For microarray sample processing, 150 ng of total RNA was used to generate singlestranded DNA for hybridization to Human Gene 1.0 ST arrays (Affymetrix, Santa Clara, CA, USA) following manufacturer's protocol. Affymetrix analysis was performed using 28,830 probes. The probe level intensity was analyzed for quality and normalized. Linear model-based limma software was used to evaluate differences between genetic expression in the NSA and adherent GBM cells [41].
All animal work was approved by the Institutional Animal Care and Use Committee (IACUC) at the University of Florida. Non-obese diabetic/severe combined immunodeficient (NOD/SCID) female mice 8-10 weeks of age were anesthetized and received a flank subcutaneous injection of 1×106 cultured L0 GBM cells in 200 µl of medium and 100 µl of Matrigel (BD). The cells had been passaged in either NSA or adherent conditions for at least 5 passages prior to xenotransplantation. The animals were divided into two equal groups based on receipt of cells from NSA (n=5) or those from adherent culture (n=5). The animals were monitored for tumor growth and were euthanized once tumors reached 1.5 cm in size. A Kaplan-Meier survival analysis was performed, ratio generated for the NSA and laminin group, and the 95% confidence interval (CI) reported to determine the relationship between type of tumor cell culture and period of survival.
After establishing GBM cell lines using the NSA, cells were cultured using either the NSA or adherent conditions. Changes in cell growth measured by the neurosphere assay have been shown to reflect changes in the TIC compartment [42]. GBM tumor neurosphere formation in culture has been found to correlate with poorer patient outcomes [43]. Moreover, the rate of GBM tumor cell growth in culture (cellular fold expansion) has been associated with disease progression [44]. For example, forcing GBM TICs to differentiate has been shown to significantly reduce cell growth in vitro and in vivo [44]. Therefore, the first comparison between the NSA and laminin culture conditions was cellular fold expansion over time.
The cells were passaged every 4-10 days depending on the cell line. The NSA and laminin cells were passaged simultaneously for each line for fair comparison (n=4 in each group representing biological replicates of different cell lines). Cells were counted at every passage by enumerating plated dissociated cells in solution, and expansion curves for the NSA and adherent culture conditions were not different (Student's t test, P=0.24) (Fig 1). These results demonstrate that the expansion of cell lines is equivalent between the two culture techniques over time.
Since specific and exclusive reliable molecular or cellular markers of neural or TICs do not exist, these cells were defined with functional criteria. Included in these criteria is clonogenic capacity, or the ability of one founder cell to create a colony consisting of TICs and more differentiated cells. The clonal frequency of GBM cells was tested in vitro with the limiting dilution assay [40]. For the adherent culture, cells were grown in 96 well plates coated with laminin with dilutions of 1-10 cells/well. The wells that at least 75% confluent were considered to have clonogenic cells. For the NSA, cells were also grown in 96-well plates. Wells with clonogenic cells were considered to be those where the number of neurospheres was equal to or greater than the number of cells plated. Using a standard calculation for clonogenic frequency [40] (by taking the negative log of the proportion of empty wells) the clonal frequency was generated for each condition. For adherent cultures the clonal frequency was 5.2% or 1 cell out of 16.4 to 22.2 cells, compared to 4.5% or 1 cell out of 16.6 to 27.4 cells for the NSA (P=0.9, n=7 each group representing two different cell lines and technical replicates of the experiments) (Electronic Supplementary Fig. 1). These results are similar to the reported clonal frequency of GBM cell lines grown in the NSA [13, 45]. Therefore, GBM cells do not demonstrate a difference in clonogenic capacity, a functional stem cell quality, when grown in the NSA compared to laminin culture conditions.
To further support proliferative profile similarities between NSA and laminin assays we compared the expression of markers such as Nestin, Ki67, and MCM2. The cells were fixed and stained with antibodies against Nestin, Ki67, and MCM2. The NSA and adherent cells had no significant differences in Nestin (P=0.52), Ki67 (P=0.22), or MCM2 (P=0.51) (Electronic Supplementary Figs. 2, 3). The figures depict percent change of the geometric mean of stained cells compared to unstained cells (Fig. 2).
GBM TICs are also characterized by being relatively undifferentiated, similar to somatic stem cells. Hence, to evaluate the ability to maintain multipotency in each growth condition, expression of differentiation markers was tested. Cells were tested for GFAP (astrocytic differentiaton) and beta tubulin III (neuronal differentiation) (Electronic Supplementary Figs. 2, 3) [21]. The geometric mean of the laminin grown cells is shown as a percentage of the geometric mean of the NSA grown cells. No statistically significant difference was found in differentiation markers GFAP (P=0.44) or beta tubulin III (P=0.18) between NSA and laminin cells (Fig. 3).
Next, the cells from three different lines were tested for expression of markers known to be expressed in TIC. Four proteins were found to be significantly up regulated in the laminin culture conditions: (1) CD44, (2) CD133, (3) CD49f, and (4) Mcl-1 (Fig. 4). These proteins were investigated due to correlation with GBM prognosis (CD44) [46], marker of GBM stem-like cells (CD133) [14], marker of stem-like cells in other types of cancer (CD49) [47] and marker of apoptotic dysregulation in GBM cells (Mcl-1) [48, 49]. However, the up regulation was not seen in all lines tested. In GB:A1, no changes in any of the proteins were seen; while GB:A3 saw an up regulation of CD44 and CD49f. Up regulation of the 4 proteins was seen in the GB:A2 line. Overall, no consistent changes in protein expression were seen among the three lines tested; however, a few individual differences were identified (Fig. 5).
Altogether, GBM cells grown in the NSA and laminin growth conditions did not demonstrate differences in proportion of stem-like TICs based on proliferative and differentiation marker expression.
Previous studies demonstrated increased apoptosis in cells grown with the NSA compared to cells grown in adherent conditions [38, 39]. However, cell death is a heterogeneous process involving multiple and sometimes overlapping pathways [50]. Therefore, we used multiple apoptosis assays to quantify the difference in cell dying and death between cells in the NSA and laminin growth conditions.
Cell death was measured at the time of passage by counting the number of cells positive for trypan blue during the cell counts. Cell death between the two groups was the same (Student's t test, P=0.7, n=9 representing two cell lines and technical replicates of the experiments) (Fig. 6). Cells were also analyzed for markers of death, late and early phases of apoptosis. Initially dead cells were identified using either PI or DAPI to stain cells with compromised cell membranes [51, 52]. Similar to the trypan blue data, no statistical difference was found between NSA or laminin cells in PI (P=0.27) (Electronic Supplementary Fig. 4) or DAPI staining (P=0.93) (Electronic Supplementary Fig. 5).
Subsequently, late, mid and early markers of apoptosis were tested. Annexin V, a marker of late apoptosis, binds phosphatidylserine which is normally confined to the inner plasma membrane, but becomes exposed with apoptosis [53]. Percentage of cells expressing annexin V in laminin cells was 7.6±1.8% compared to 10.1±1.5% in NSA cells (P=0.32) (Electronic Supplementary Fig. 6).
The annexin V cell experiments were followed by testing for activated caspase 3 (activated death protease that catalyzes the cleavage of key cellular proteins [54, 55, 56] expression in live and fixed cells. Using a caspase 3 inhibitor conjugated to a fluorochrome (PE), activated caspase 3 was quantified in live cells. The staining was equivalent between NSA (5.9±1.0%) and laminin cells (7.2±1.2%, P=0.41) (Electronic Supplementary Fig. 7A-D). Conversely, measuring the amount of antibody staining activated caspase 3 in fixed cells, the laminin cultured cells demonstrated a trend for increased immunoreactivity (4.4±0.6%) when compared to NSA culture (2.2±0.6%, P=0.026) (Electronic Supplementary Fig. 7E-H).
Live cells were tested for DiIC, identifying apoptotic cells as those without DiIC staining. Cyanine dyes, such as DiIC, penetrate eukaryotic cell membranes and accumulate in the mitochondria with active membrane potentials. When this potential is disrupted (early sign of apoptosis), there is less DiIC staining. No statistically significant difference between the NSA and laminin cells was found (P=0.55) (Electronic Supplementary Fig. 8).
Based on multiple markers, no difference exists in terms of apoptosis between GBM cells grown in NSA or laminin culture conditions (Fig. 7, Electronic Supplementary Fig. 9).
GBM cells were grown in the NSA (n=6) or adherent (n=6, representing two cell lines and technical replicates of the experiments) culture conditions and were collected for RNA isolation and affymetrix analysis. First, genetic expression as a ratio of laminin and NSA cells that varied by a log fold change. Comparing all of the laminin and NSA grown GBM cells revealed laminin cells had 23 upregulated genes including CD44 and 7 downregulated genes. However, these values included changes that were not statistically significant. When the data were evaluated for genetic expression changes that were significant using false discovery rate measures to avoid type I errors, no difference was found between the laminin and NSA GBM cells (Table 1). In conclusion, using affymetrix analysis, no significant genetic differences were found between GBM cells grown in laminin and the NSA, supporting the phenotypic data.
After demonstrating GBM cells maintained stem cell characteristics when grown in the two different culture conditions, tumorigenicity was compared in a xenotransplant model. Tumorigenicity is a critical capability of TICs, and ability to maintain tumorigenic cells is important for a culture condition to be useful. NOD/SCID mice received a flank subcutaneous injection of 1×106 cultured L0 GBM cells. The cells had been passaged in either NSA or adherent conditions for at least 5 passages prior to xenotransplantation. The animals were divided into two equal groups based on receipt of cells from NSA (n=5) or those from adherent culture (n=5). The growth of the tumors (Electronic Supplementary Fig. 10) and survival analysis was the same for hGBM cells grown in the NSA (median survival 78 days) and those grown with laminin (median survival 71 days; ratio, 1.099; 95% CI, 0.8-1.4) (Fig. 8). GBM cells grown in the NSA and laminin culture conditions demonstrated similar ability to initiate and propagate tumor in a xenotransplant model.
The NSA is the current standard for identifying and maintaining brain TICs in culture [26]. However, this assay has notable limitations including variable composition of cells [29, 30] and overestimation of proportion of NSCs [31]. To overcome these limitations, modifications to the NSA have been proposed, including the addition of laminin to grow cells flat. Some advantages of adherent culture conditions suggested by these reports include a more pure stem cell population, decreased differentiation, increased tumorigenicity, and decreased cellular apoptosis [39].
Recent reports claim that cells from NSA spheroids have increased differentiation in expansion conditions, although this finding was not statistically analyzed [39]. As TICs differentiate, they lose their stem cell characteristics such as self-renewal, generation of a large number of progeny and multipotency [57]. Consequently, TIC culture conditions should maintain relatively undifferentiated cells that are multipotent (retain the ability to differentiate when exposed to a differentiation agent). In our study, the NSA and laminin cells were not different with regard to staining for nestin (relatively undifferentiated cells), GFAP (astrocyte differentiation) or beta tubulin III (neuronal differentiation) in cell expansion conditions. The differences between our results and other reports may be due to overgrowth of the neurospheres in other studies, resulting in increased cellular differentiation [58]. The two culture conditions did demonstrate variability in protein markers. This may be due to the variable growth of different lines in the two distinct culture conditions, and will require further study.
In addition to the purity of the TIC population, increased tumorigenicity was described as an advantage of laminin-cultured cells. Laminin has also been implicated in GBM invasion and migration based on in vitro migration analyses [59, 60]. In our subcutaneous xenograft model, GBM cells grown in laminin did not show increased tumorigenicity or invasion. The animals implanted with NSA and laminin-cultured cells had similar tumor growth and survival times. Moreover, using an in vitro limiting dilution assay, the two culture conditions demonstrated similar clonogenic potential of the GBM cell lines. However, conclusions from these experiments are limited due to use of a limited number of cell lines, and the need to repeat the limiting dilution assay and survival experiments with an intracranial xenograft model.
The GBM cells grown in NSA and adherent culture conditions did not show differences in cell death or dying as quantified by apoptotic markers. The previous culture studies demonstrated increased apoptosis in cells grown with NSA compared to cells grown in adherent conditions [38, 39]. This literature demonstrated increased apoptosis in NSA cells using annexin V and the terminal deoxynucleotidyl transferase dUTP nick end labeling (detecting fragmented DNA) assays [34, 39]. The decreased apoptosis with laminin was hypothesized to be due to laminin preventing cellular apoptosis that is secondary to detachment from the extracellular membrane [34]. Cell death is a heterogeneous process involving multiple and sometimes overlapping pathways [50]. Therefore, we used multiple apoptosis assays to quantify the difference in the cell dying process and cell death between cells in the NSA and laminin growth conditions. No significant difference in apoptosis or cell death was found between NSA and laminin cultured cells. The previously reported differences in apoptosis are limited by testing of only one or two apoptosis measures.
In conclusion, based on functional criteria such as our long-term proliferation data, cells grown in the NSA and laminin culture conditions have no differences in the proportion of TICs and progenitors. Laminin grown cells also do not show increased clonal frequency in vitro or increased tumorigenicity in a xenograft model compared to the NSA. Furthermore, cells grown in the NSA and laminin have no differences in cell death or any significant differences in genetic expression. Overall, these data show no advantage of the laminin culture method to the NSA.
Developing novel and effective therapy for GBM is challenging due to tumor heterogeneity, robustness and imperfect tumor models. The development of alternative culture techniques for establishing cell lines is a useful addition to the existing tools for the study of GBM. Although potentially more convenient for certain applications, GBM cells grown in adherent conditions are not significantly different from cells grown with the traditional NSA.
Figures and Tables
Fig. 1
The number of cells obtained in vitro over multiple passages of glioblastoma cell lines grown in neurosphere assay (NSA) and adherent culture conditions (n=4 each group). The fold expansion over time in the two different conditions was not statistically different (P=0.24).
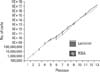
Fig. 2
The geometric mean of markers of proliferation markers nestin (n=4, P=0.52), Ki67 (n=3, P=0.22), and MCM2 (n=7, P=0.51). The mean and standard error are shown in the graphs. NSA, neurosphere assay.
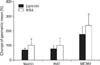
Fig. 3
The geometric mean of markers of differentiation including glial fibrillary acidic protein (GFAP) (n=6, P=0.44) and beta tubulin III (n=6, P=0.18) of laminin-grown cells compared to neurosphere assay (NSA) grown cells by flow cytometry. The mean and standard error are shown in the graphs.
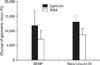
Fig. 4
(A-D) The geometric mean of protein expression in various glioblastoma cell lines in laminin (L) and neurosphere assay (N) conditions.
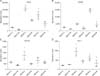
Fig. 5
Graph depicting mean geometric mean values of protein expression in laminin versus neurosphere assay (NSA) grown glioblastoma cells (n=3). EGFR, epidermal growth factor receptor. *Statistically significant.
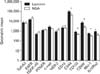
Fig. 6
Percent of cells positive for trypan blue denoting cell death at the time of trypsinization of neurospheres or cells grown on laminin (n=9). Standard error is shown with the error bars (P=0.7). NSA, neurosphere assay.
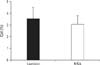
Fig. 7
Summary of apoptosis markers including propidium iodide (PI) (n=4), annexin V (n=4), activated caspase 3 (n=6), 4',6-diamidino-2-phenylindole (DAPI) (n=7), and 1,1',3,3,3',3'-hexamethylindodicarbocyanine iodide (DiIC) (n=5) by flow cytometry depicting percent of cells positive in neurosphere assay (NSA) and laminin grown glioblastoma cells. P-value, not significant for all comparisons. The mean and standard error are shown in the graph.
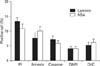
Fig. 8
Kaplan-Meier demonstrating similar survival of non-obese diabetic/severe combined immunodeficient mice after implantation of glioblastoma cells grown in either neurosphere assay (NSA) and laminin culture conditions (n=5 in each group). Mice were euthanized once tumors reached 1.5 cm in any dimension.
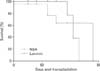
Table 1
Affymetrix analysis
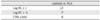
Laminin vs. NSA | |
---|---|
Log FC ≥ 1 | 23 |
Log FC ≤ -1 | 7 |
FDR ≤ 0.05 | 0 |
Affymetrix analysis with number of probes shown. Laminin and neurosphere assay (NSA) glioblastoma (GBM) cells were compared. For statistically significant differences, false discovery rate (FDR) was set at ≤0.05 and no genetic expression differences were found between laminin and NSA GBM cells. FC, fold change.
References
1. Reynolds BA, Weiss S. Generation of neurons and astrocytes from isolated cells of the adult mammalian central nervous system. Science. 1992; 255:1707–1710.
2. Morshead CM, Reynolds BA, Craig CG, McBurney MW, Staines WA, Morassutti D, Weiss S, van der Kooy D. Neural stem cells in the adult mammalian forebrain: a relatively quiescent subpopulation of subependymal cells. Neuron. 1994; 13:1071–1082.
3. Weiss S, Dunne C, Hewson J, Wohl C, Wheatley M, Peterson AC, Reynolds BA. Multipotent CNS stem cells are present in the adult mammalian spinal cord and ventricular neuroaxis. J Neurosci. 1996; 16:7599–7609.
4. Hamilton A. The division of the differentiated cells in the central nervous system of the white rat. J Comp Neurol. 1901; 11:297–320.
5. Kershman J. The medulloblast and the medulloblastoma: a study of human embryos. Arch Neurol Psychiatr. 1938; 40:937–967.
6. Bryans WA. Mitotic activity in the brain of the adult rat. Anat Rec. 1959; 133:65–73.
7. Altman J, Das GD. Autoradiographic and histological studies of postnatal neurogenesis. I. A longitudinal investigation of the kinetics, migration and transformation of cells incorporating tritiated thymidine in neonate rats, with special reference to postnatal neurogenesis in some brain regions. J Comp Neurol. 1966; 126:337–389.
8. Dacey ML, Wallace RB. Postnatal neurogenesis in the feline cerebellum: a structural-functional investigation. Acta Neurobiol Exp (Wars). 1974; 34:253–263.
9. Kaplan MS, Hinds JW. Neurogenesis in the adult rat: electron microscopic analysis of light radioautographs. Science. 1977; 197:1092–1094.
10. Messier B, Leblond CP, Smart I. Presence of DNA synthesis and mitosis in the brain of young adult mice. Exp Cell Res. 1958; 14:224–226.
11. Paton JA, Nottebohm FN. Neurons generated in the adult brain are recruited into functional circuits. Science. 1984; 225:1046–1048.
12. Goldman SA, Nottebohm F. Neuronal production, migration, and differentiation in a vocal control nucleus of the adult female canary brain. Proc Natl Acad Sci U S A. 1983; 80:2390–2394.
13. Galli R, Binda E, Orfanelli U, Cipelletti B, Gritti A, De Vitis S, Fiocco R, Foroni C, Dimeco F, Vescovi A. Isolation and characterization of tumorigenic, stem-like neural precursors from human glioblastoma. Cancer Res. 2004; 64:7011–7021.
14. Singh SK, Hawkins C, Clarke ID, Squire JA, Bayani J, Hide T, Henkelman RM, Cusimano MD, Dirks PB. Identification of human brain tumour initiating cells. Nature. 2004; 432:396–401.
15. Fang D, Nguyen TK, Leishear K, Finko R, Kulp AN, Hotz S, Van Belle PA, Xu X, Elder DE, Herlyn M. A tumorigenic subpopulation with stem cell properties in melanomas. Cancer Res. 2005; 65:9328–9337.
16. Ricci-Vitiani L, Lombardi DG, Pilozzi E, Biffoni M, Todaro M, Peschle C, De Maria R. Identification and expansion of human colon-cancer-initiating cells. Nature. 2007; 445:111–115.
17. Eramo A, Lotti F, Sette G, Pilozzi E, Biffoni M, Di Virgilio A, Conticello C, Ruco L, Peschle C, De Maria R. Identification and expansion of the tumorigenic lung cancer stem cell population. Cell Death Differ. 2008; 15:504–514.
18. Bonnet D, Dick JE. Human acute myeloid leukemia is organized as a hierarchy that originates from a primitive hematopoietic cell. Nat Med. 1997; 3:730–737.
19. Huntly BJ, Gilliland DG. Leukaemia stem cells and the evolution of cancer-stem-cell research. Nat Rev Cancer. 2005; 5:311–321.
20. Vescovi AL, Galli R, Reynolds BA. Brain tumour stem cells. Nat Rev Cancer. 2006; 6:425–436.
21. Ignatova TN, Kukekov VG, Laywell ED, Suslov ON, Vrionis FD, Steindler DA. Human cortical glial tumors contain neural stemlike cells expressing astroglial and neuronal markers in vitro. Glia. 2002; 39:193–206.
22. Bao S, Wu Q, McLendon RE, Hao Y, Shi Q, Hjelmeland AB, Dewhirst MW, Bigner DD, Rich JN. Glioma stem cells promote radioresistance by preferential activation of the DNA damage response. Nature. 2006; 444:756–760.
23. Liu G, Yuan X, Zeng Z, Tunici P, Ng H, Abdulkadir IR, Lu L, Irvin D, Black KL, Yu JS. Analysis of gene expression and chemoresistance of CD133+ cancer stem cells in glioblastoma. Mol Cancer. 2006; 5:67.
24. Eramo A, Ricci-Vitiani L, Zeuner A, Pallini R, Lotti F, Sette G, Pilozzi E, Larocca LM, Peschle C, De Maria R. Chemotherapy resistance of glioblastoma stem cells. Cell Death Differ. 2006; 13:1238–1241.
25. Rich JN. Cancer stem cells in radiation resistance. Cancer Res. 2007; 67:8980–8984.
26. Chaichana K, Zamora-Berridi G, Camara-Quintana J, Quiñones-Hinojosa A. Neurosphere assays: growth factors and hormone differences in tumor and nontumor studies. Stem Cells. 2006; 24:2851–2857.
27. Lee J, Kotliarova S, Kotliarov Y, Li A, Su Q, Donin NM, Pastorino S, Purow BW, Christopher N, Zhang W, Park JK, Fine HA. Tumor stem cells derived from glioblastomas cultured in bFGF and EGF more closely mirror the phenotype and genotype of primary tumors than do serum-cultured cell lines. Cancer Cell. 2006; 9:391–403.
28. De Witt Hamer PC, Van Tilborg AA, Eijk PP, Sminia P, Troost D, Van Noorden CJ, Ylstra B, Leenstra S. The genomic profile of human malignant glioma is altered early in primary cell culture and preserved in spheroids. Oncogene. 2008; 27:2091–2096.
29. Arsenijevic Y, Weiss S, Schneider B, Aebischer P. Insulin-like growth factor-I is necessary for neural stem cell proliferation and demonstrates distinct actions of epidermal growth factor and fibroblast growth factor-2. J Neurosci. 2001; 21:7194–7202.
30. Caldwell MA, He X, Wilkie N, Pollack S, Marshall G, Wafford KA, Svendsen CN. Growth factors regulate the survival and fate of cells derived from human neurospheres. Nat Biotechnol. 2001; 19:475–479.
31. Reynolds BA, Rietze RL. Neural stem cells and neurospheres: reevaluating the relationship. Nat Methods. 2005; 2:333–336.
32. Singec I, Knoth R, Meyer RP, Maciaczyk J, Volk B, Nikkhah G, Frotscher M, Snyder EY. Defining the actual sensitivity and specificity of the neurosphere assay in stem cell biology. Nat Methods. 2006; 3:801–806.
33. Louis SA, Rietze RL, Deleyrolle L, Wagey RE, Thomas TE, Eaves AC, Reynolds BA. Enumeration of neural stem and progenitor cells in the neural colony-forming cell assay. Stem Cells. 2008; 26:988–996.
34. Hall PE, Lathia JD, Caldwell MA, Ffrench-Constant C. Laminin enhances the growth of human neural stem cells in defined culture media. BMC Neurosci. 2008; 9:71.
35. Pollard SM, Conti L, Sun Y, Goffredo D, Smith A. Adherent neural stem (NS) cells from fetal and adult forebrain. Cereb Cortex. 2006; 16:Suppl 1. i112–i120.
36. Conti L, Pollard SM, Gorba T, Reitano E, Toselli M, Biella G, Sun Y, Sanzone S, Ying QL, Cattaneo E, Smith A. Niche-independent symmetrical self-renewal of a mammalian tissue stem cell. PLoS Biol. 2005; 3:e283.
37. Sun Y, Pollard S, Conti L, Toselli M, Biella G, Parkin G, Willatt L, Falk A, Cattaneo E, Smith A. Long-term tripotent differentiation capacity of human neural stem (NS) cells in adherent culture. Mol Cell Neurosci. 2008; 38:245–258.
38. Fael Al-Mayhani TM, Ball SL, Zhao JW, Fawcett J, Ichimura K, Collins PV, Watts C. An efficient method for derivation and propagation of glioblastoma cell lines that conserves the molecular profile of their original tumours. J Neurosci Methods. 2009; 176:192–199.
39. Pollard SM, Yoshikawa K, Clarke ID, Danovi D, Stricker S, Russell R, Bayani J, Head R, Lee M, Bernstein M, Squire JA, Smith A, Dirks P. Glioma stem cell lines expanded in adherent culture have tumor-specific phenotypes and are suitable for chemical and genetic screens. Cell Stem Cell. 2009; 4:568–580.
40. Hu Y, Smyth GK. ELDA: extreme limiting dilution analysis for comparing depleted and enriched populations in stem cell and other assays. J Immunol Methods. 2009; 347:70–78.
41. Smyth GK, Michaud J, Scott HS. Use of within-array replicate spots for assessing differential expression in microarray experiments. Bioinformatics. 2005; 21:2067–2075.
42. Deleyrolle LP, Ericksson G, Morrison BJ, Lopez JA, Burrage K, Burrage P, Vescovi A, Rietze RL, Reynolds BA. Determination of somatic and cancer stem cell self-renewing symmetric division rate using sphere assays. PLoS One. 2011; 6:e15844.
43. Laks DR, Masterman-Smith M, Visnyei K, Angenieux B, Orozco NM, Foran I, Yong WH, Vinters HV, Liau LM, Lazareff JA, Mischel PS, Cloughesy TF, Horvath S, Kornblum HI. Neurosphere formation is an independent predictor of clinical outcome in malignant glioma. Stem Cells. 2009; 27:980–987.
44. Piccirillo SG, Reynolds BA, Zanetti N, Lamorte G, Binda E, Broggi G, Brem H, Olivi A, Dimeco F, Vescovi AL. Bone morphogenetic proteins inhibit the tumorigenic potential of human brain tumour-initiating cells. Nature. 2006; 444:761–765.
45. Inagaki A, Soeda A, Oka N, Kitajima H, Nakagawa J, Motohashi T, Kunisada T, Iwama T. Long-term maintenance of brain tumor stem cell properties under at non-adherent and adherent culture conditions. Biochem Biophys Res Commun. 2007; 361:586–592.
46. Wei KC, Huang CY, Chen PY, Feng LY, Wu TW, Chen SM, Tsai HC, Lu YJ, Tsang NM, Tseng CK, Pai PC, Shin JW. Evaluation of the prognostic value of CD44 in glioblastoma multiforme. Anticancer Res. 2010; 30:253–259.
47. Pece S, Tosoni D, Confalonieri S, Mazzarol G, Vecchi M, Ronzoni S, Bernard L, Viale G, Pelicci PG, Di Fiore PP. Biological and molecular heterogeneity of breast cancers correlates with their cancer stem cell content. Cell. 2010; 140:62–73.
48. Day BW, Stringer BW, Spanevello MD, Charmsaz S, Jamieson PR, Ensbey KS, Carter JC, Cox JM, Ellis VJ, Brown CL, Walker DG, Inglis PL, Allan S, Reynolds BA, Lickliter JD, Boyd AW. ELK4 neutralization sensitizes glioblastoma to apoptosis through downregulation of the anti-apoptotic protein Mcl-1. Neuro Oncol. 2011; 13:1202–1212.
49. Rahaman SO, Harbor PC, Chernova O, Barnett GH, Vogelbaum MA, Haque SJ. Inhibition of constitutively active Stat3 suppresses proliferation and induces apoptosis in glioblastoma multiforme cells. Oncogene. 2002; 21:8404–8413.
50. Galluzzi L, Aaronson SA, Abrams J, Alnemri ES, Andrews DW, Baehrecke EH, Bazan NG, Blagosklonny MV, Blomgren K, Borner C, Bredesen DE, Brenner C, Castedo M, Cidlowski JA, Ciechanover A, Cohen GM, De Laurenzi V, De Maria R, Deshmukh M, Dynlacht BD, El-Deiry WS, Flavell RA, Fulda S, Garrido C, Golstein P, Gougeon ML, Green DR, Gronemeyer H, Hajnoczky G, Hardwick JM, Hengartner MO, Ichijo H, Jaattela M, Kepp O, Kimchi A, Klionsky DJ, Knight RA, Kornbluth S, Kumar S, Levine B, Lipton SA, Lugli E, Madeo F, Malomi W, Marine JC, Martin SJ, Medema JP, Mehlen P, Melino G, Moll UM, Morselli E, Nagata S, Nicholson DW, Nicotera P, Nunez G, Oren M, Penninger J, Pervaiz S, Peter ME, Piacentini M, Prehn JH, Puthalakath H, Rabinovich GA, Rizzuto R, Rodrigues CM, Rubinsztein DC, Rudel T, Scorrano L, Simon HU, Steller H, Tschopp J, Tsujimoto Y, Vandenabeele P, Vitale I, Vousden KH, Youle RJ, Yuan J, Zhivotovsky B, Kroemer G. Guidelines for the use and interpretation of assays for monitoring cell death in higher eukaryotes. Cell Death Differ. 2009; 16:1093–1107.
51. Troiano L, Ferraresi R, Lugli E, Nemes E, Roat E, Nasi M, Pinti M, Cossarizza A. Multiparametric analysis of cells with different mitochondrial membrane potential during apoptosis by polychromatic flow cytometry. Nat Protoc. 2007; 2:2719–2727.
52. Mazzini G, Ferrari C, Erba E. Dual excitation multi- fluorescence flow cytometry for detailed analyses of viability and apoptotic cell transition. Eur J Histochem. 2003; 47:289–298.
53. Martin K, Akinwunmi J, Rooprai HK, Kennedy AJ, Linke A, Ognjenovic N, Pilkington GJ. Nonexpression of CD15 by neoplastic glia: a barrier to metastasis? Anticancer Res. 1995; 15:1159–1166.
54. Porter AG, Jänicke RU. Emerging roles of caspase-3 in apoptosis. Cell Death Differ. 1999; 6:99–104.
55. Jänicke RU, Ng P, Sprengart ML, Porter AG. Caspase-3 is required for alpha-fodrin cleavage but dispensable for cleavage of other death substrates in apoptosis. J Biol Chem. 1998; 273:15540–15545.
56. Jänicke RU, Sprengart ML, Wati MR, Porter AG. Caspase-3 is required for DNA fragmentation and morphological changes associated with apoptosis. J Biol Chem. 1998; 273:9357–9360.
57. Potten CS, Loeffler M. Stem cells: attributes, cycles, spirals, pitfalls and uncertainties. Lessons for and from the crypt. Development. 1990; 110:1001–1020.
58. Gritti A, Galli R, Vescovi AL. Cultures of stem cells of the central nervous system. In : Fedoroff S, Richardson A, editors. Protocols for Neural Cell Culture. 3rd ed. Totowa: Humana Press;2001. p. 173–198.
59. Mahesparan R, Tysnes BB, Read TA, Enger PO, Bjerkvig R, Lund-Johansen M. Extracellular matrix-induced cell migration from glioblastoma biopsy specimens in vitro. Acta Neuropathol. 1999; 97:231–239.
60. Ziu M, Schmidt NO, Cargioli TG, Aboody KS, Black PM, Carroll RS. Glioma-produced extracellular matrix influences brain tumor tropism of human neural stem cells. J Neurooncol. 2006; 79:125–133.
Electronic Supplementary Materials
Supplementary data including 10 figures can be found with this article online at http://www.acbjournal.org/src/sm/acb-48-25-s001.pdf.
Supplementary Fig. 1
(A) Limiting dilution assay of cells grown using laminin. Determination of clonogenic capacity was based on ability of cell grown on laminin to fill a well. Full well shown. (B) Limiting dilution assay of cells grown using the neurosphere assay. Determination of clonogenic capacity was based on ability of cells to generate spheres. Tumor neurospheres shown.
Supplementary Fig. 2
(A-F) Representative flow cytometry plots for marker expression using neurosphere assay (NSA) cells. Positive cells were identified by applying a gate created to separate positive and negative staining cells from the controls. (B) Ki67 staining of NSA cells. (C) Nestin staining of NSA cells. (D) Glial fibrillary acidic protein (GFAP) staining of NSA cells. (E) Beta tubulin III staining of NSA cells. (F) MCM2 staining of NSA cells.
Supplementary Fig. 3
(A-F) Representative flow cytometry plots for marker expression using laminin cells. Positive cells were identified by applying a gate created to separate positive and negative staining cells from the controls. (B) Ki67 staining of laminin cells. (C) Nestin staining of laminin cells. (D) Glial fibrillary acidic protein (GFAP) staining of laminin cells. (E) Beta tubulin III staining of laminin cells. (F) MCM2 staining of laminin cells.
Supplementary Fig. 4
Representative flow cytometry plots from propidium iodide (PI) experiments. (A) Laminin cells with no PI are shown. Using the PI fluorescence of the cells, a gate was identified (line) to separate PI positive and PI negative cells. Applying this gate to the cells stained with PI (B) 3.21% of the cells were found to be PI positive/dead. (C) Neurosphere assay (NSA) cells with no PI are shown. Using the PI fluorescence of the cells, a gate was identified (line) to separate PI positive and PI negative cells. Applying this gate to the cells stained with PI (D) 1.59% of the cells were found to be PI positive/dead.
Supplementary Fig. 5
Representative flow cytometry plots from DAPI experiments. (A) Laminin cells with no DAPI are shown. Using the DAPI fluorescence of the cells, a gate was identified (line) to separate DAPI positive and DAPI negative cells. Applying this gate to the cells stained with DAPI (B) 1.02% of the cells were found to be DAPI positive/dead. (C) Neurosphere assay (NSA) cells with no DAPI are shown. Using the DAPI fluorescence of the cells, a gate was identified (line) to separate DAPI positive and DAPI negative cells. Applying this gate to the cells stained with DAPI (D) 1.14% of the cells were found to be DAPI positive/dead.
Supplementary Fig. 6
Flow cytometry plots demonstrating annexin V and propidium iodide (PI) staining. (A) Laminin cells stained with PI only to identify dead cells. Using the flurorescence of pacific blue (the fluorochrome conjugated to the annexin V antibody) of these cells, a gate was identified (lines) to separate live/dead cells (PI-/+) and annexin V -/+ cells (pacific blue -/+). This gate was applied to cells stained with PI and an antibody to annexin V conjugated to pacific blue (B). 6.52% of the cells were positive for annexin V and apoptotic. 0.87% of the cells were positive for annexin V but also positive for PI and therefore already dead. (C) Neurosphere assay (NSA) cells stained with PI only to identify dead cells. Using the flurorescence of pacific blue (the fluorochrome conjugated to the annexin V antibody) of these cells, a gate was identified (lines) to separate live/dead cells (PI -/+) and annexin V -/+ cells (pacific blue -/+). This gate was applied to cells stained with PI and an antibody to annexin V conjugated to pacific blue (D). 12.6% of the cells were positive for annexin V and apoptotic. 0.81% of the cells were positive for annexin V but also positive for PI and therefore already dead.
Supplementary Fig. 7
Flow cytometry plots demonstrating caspase activity staining glioblastoma cells grown in neurosphere assay (NSA) and laminin culture conditions. (A) Live cells without caspase 3 inhibitor. Using the PE (fluorochrome conjugated to the caspase 3 inhibitor) fluorescence of cells, a gate was identified (line) to separate PE -/+ cells. Applying this gate to cells with caspase 3 inhibitor (B), 2.16% of laminin cells were found to express activated caspase 3. Similarly, a gate was chosen using live NSA control cells (C). Applying this gate to cells with caspase 3 inhibitor (D), 1.67% of cells were found to express activated caspase 3. In experiments with fixed cells, using the fluorescence of pacific blue (fluorochrome conjugated to the caspase 3 antibody) of the cells with no antibody, a gate was identified to separate pacific blue -/+ cells (E, G). Applying these gates to cells with the antibody to activated caspase 3, 10.8% of laminin and 4.63% of NSA cells were found to express activated caspase 3 (F, H).
Supplementary Fig. 8
Flow cytometry plots showing DiIC staining in laminin and neurosphere assay (NSA) grown cells. Cells stained with DAPI only were used to chose a gate (lines) to separate live/dead cells (DAPI -/+) and 1,1',3,3,3',3'-hexamethylindodicarbo-cyanine iodide (DiIC) -/+ (AF633 fluorochrome -/+) (A, C). These gates were applied to cells stained with DAPI and DiIC conjugated to AF633 (B, D). 86% of laminin grown cells and 97% of NSA grown cells were positive for DiIC (non-apoptotic).
Supplementary Fig. 9
Summary of apoptosis experiments including propidium iodide (PI), annexin V, activated caspase 3, 4',6-diamidino-2-phenylindole (DAPI) and 1,1',3,3,3',3'-hexamethylindodicarbocyanine iodide (DiIC). No statistical differences were found between cells grown in neurosphere assay (NSA) and laminin culture conditions.