Abstract
Pulmonary arterial hypertension (PAH) is associated with structural alterations of lung vasculature. PAH is still a devastating disease needing an aggressive therapeutic approach. Despite the therapeutic potential of human umbilical cord mesenchymal stem cells (MSCs), the molecular parameters to define the stemness remain largely unknown. Using high-density oligonucleotide microarrays, the differential gene expression profiles between a fraction of mononuclear cells of human umbilical cord blood (UCB) and its MSC subpopulation were obtained. Of particular interest was a subset of 46 genes preferentially expressed at 7-fold or higher in the group treated with human UCB-MSCs. This subset contained numerous genes involved in the inflammatory response, immune response, lipid metabolism, cell adhesion, cell migration, cell differentiation, apoptosis, cell growth, transport, cell proliferation, transcription, and signal transduction. Our results provide a foundation for a more reproducible and reliable quality control using genotypic analysis for the definition of human UCB-MSCs. Therefore, our results will provide a basis for studies on molecular mechanisms controlling the core properties of human MSCs.
Pulmonary artery hypertension (PAH) is a progressive disease, with an estimated median survival of 2.8 years from the time of diagnosis to death [1]. PAH has a complex pathogenesis and is characterized by arteriolar wall remodeling, elevated pulmonary artery pressure, and right ventricular (RV) hypertrophy [2]. The increased pulmonary vascular resistance and excessive proliferation of endothelial cells in the pulmonary artery lead to pulmonary vascular remodeling [3, 4, 5], with widespread loss of the pulmonary microvasculature carrying blood from the heart to the lungs [6]. Although current treatments may prolong and improve the quality of life of patients, the long-term outcome of this disorder is lacking.
Mesenchymal stem cells (MSC) represent an archetype of multipotent somatic stem cells. MSC could differentiate into cardiomyocytes, or even cells of nonmesodermal derivation, including hepatocytes and neurons [7, 8, 9, 10, 11]. Although MSC were originally isolated from bone marrow [12, 13], similar populations have been reported in other tissues. Human MSC have now been isolated from adipose tissue [14], umbilical cord blood (UCB) [15, 16, 17, 18], and peripheral blood [19, 20]. Recently, Kogler et al. [18] described a subset of MSC derived from human CB which they called "unrestricted somatic stem cells." All of the research demonstrated that variations in conditions had a significant impact on the potential of the populations generated, even though the initial cell material could be phenotypically identical. MSC have been defined by plastic adherent growth and subsequent expansion under specific culture conditions, by a panel of nonspecific surface antigens, and by in vitro and in vivo differentiation potential [21]. However, the lack of common standards and of a precise definition of initial cell preparations remains a major obstacle for the research and applications of MSC. In the present study, MSC isolated from UCB were used to treat PAH rats, and the genome-wide expression profiles were compared. The advantage of DNA microarray analysis is that it can simultaneously evaluate changes in the relative expression of thousands of genes [22, 23, 24]. The purpose of this study was to investigate the changes of gene expression by microarray analysis after the injection of human UCB-MSCs into monocrotaline (MCT)-induced PAH rats.
Six-week-old male Sprague-Dawley rats, weighing approximately 180-220 g, were used for this study. All rats were housed in climate-controlled conditions with a 12 hour light/12 hour dark cycle, and had free access to food and water [25]. Pulmonary hypertension was induced by subcutaneous injection of 60 mg/kg MCT (Sigma Chemicals, St. Louis, MO, USA) dissolved in 0.5 N HCl solution. The rats were grouped as follows: control group (n=12), subcutaneous injection of saline (0.1 ml/kg); M group (n=12), subcutaneous injection of MCT; U group, human UCB-MSCs transfusion (n=12). Human UCB-MSCs (3×106/ml/cm2) were transfused by external jugular vein administered 1 week after MCT injection. The animals were sacrificed in 4 weeks after human UCB-MSCs transfusion. The rats were anesthetized by Zoletil 50 (30 mg/kg, Virbac, Carros Cedex, France) and Rompun (10 mg/kg, Bayer Korea Ltd., Seoul, Korea). Lung tissues were removed and immediately for microarray analysis. All protocols were approved by the Institutional Animal Care and Use Committees of the School of Medicine of Ewha Womans University (approval number 11-0169).
Human UCB-MSCs were obtained from Medipost Inc. (Biomedical Research Institute Co., Ltd., Seoul, Korea) and isolated human MSCs were expanded in culture according to the method of previous report [26]. Briefly, mononuclear cells isolated from UCB were washed, suspended in alphaminimum essential medium (Gibco-Invitrogen, Carlsbad, CA, USA) supplemented with 10% fetal bovine serum (Gibco-Invitrogen, Grand island, NY, USA). Human UCB-MSCs were adherent to plastic culture dishes during the culture period and showed spindle-shaped fibroblast-like morphology at passage 5.
Total RNA was extracted from the lung sample that stored for 24 hours at room temperature and then in the fridge (-20℃) using a PAXgene lung RNA extraction kit according to the manufacturer's instructions. Each total RNA sample (1 µg) was labeled and amplified using Universal Linkage System aRNA labeling kit (Kreatech Diagnostics, Amsterdam, Netherlands).
Each total RNA sample (100 ng) was labeled and amplified using Low Input Quick Amp labeling kit (Agilent Technologies, CA, USA). The Cy3-labeled aRNAs were resuspended in 50 µl of hybridization solution (Agilent Technologies, Santa Clara, CA, USA). After labeled aRNAs were placed on Agilent SurePrint G3 Rat GE 8X60K array (Agilent Technologies) and covered by a Gasket 8-plex slide (Agilent Technologies). The slides were hybridized for 17 hours at 65℃ oven. The hybridized slides were washed in 2× saline sodium citrate (SSC), 0.1% sodium dodecyl sulfate for 2 minutes, 1× SSC for 3 minutes, and then 0.2× SSC for 2 minutes at room temperature. The slides were centrifuged at 3,000 rpm for 20 seconds to dry.
The arrays were analyzed using an Agilent scanner with associated software. Gene expression levels were calculated with Feature Extraction v10.7.3.1 (Agilent Technologies). Relative signal intensities for each gene were generated using the Robust Multi-Array Average algorithm. The data were processed based on quantile normalization method using the GeneSpring GX 11.5.1 (Agilent Technologies). This normalization method aims to make the distribution of intensities for each array in a set of arrays the same. The normalized, and log transformed intensity values were then analyzed using GeneSpring GX 11.5.1 (Agilent Technologies). Fold change filters included the requirement that the genes be present in at least 200% of controls for up-regulated genes and lower than 50% of controls for down-regulated genes.
The expressions of 393 genes in the M treatment group were significantly different to those of the control group. M treatment showed a 1.5-fold increase in the expression of 1,996 genes (two-fold increase in the expression of 557 genes) and 1.5-fold decrease in the expressions of 1,797 genes (two-fold decrease in the expression of 563 genes) compared to the control group (Table 1). Among the up-regulated genes (Table 2), four genes (Lipase, Cytochrome P450, Acyl-CoA thioesterase 1, and Apolipoprotein C-l) were related to lipid metabolism, seven were related to transport (Aquaporin 3 [Aqp3], Rh-associated glycoprotein, Adducin 2 beta, Solute carrier family 4, Globin alpha, Solute carrier family 10, and Potassium voltage-gated channel), and fourteen were related to signal transduction (Olfactory receptor 1584 [Olr1584], Glucagon receptor, Arachidonate 15-lipoxygenase, and Neuropeptide Y receptor Y1). Galanin prepropeptide (Gal) was related to apoptosis, and arachidonate 15-lipoxygenase (Alox15b) was related to cell growth. Three genes (Hemogen [Hemgn], Zinc finger and BTB domain containing 16 [Zbtb16], Kohjirin [Chrdl1]) were related to cell differentiation, and two were related to transcription (cAMP responsive element binding protein 3-like 3 [Creb313], Nuclear receptor subfamily 1, group D, member 1 [Nr1d1]). Twenty one genes were down-regulated in the M group compared with the control group (Table 3). Among the down-regulated genes, six genes (Synaptotagmin-like 4 [Sytl4], Solute carrier family 7, member 10 [Slc7a10], Gap junction protein, beta 1 [Gjb1], Neuromedin U receptor 1 (Nmur1), Apolipoprotein L 9a [Apo19a], and Receptor [chemosensory] transporter protein 4 [Rtp4]) were related to transport, five were related to immune response (RT1 class Ib, locus S3 [RT1-S3], Apolipoprotein C-III [Apoc3], 2'-5'-oligoadenylate synthetase-like [Oasl], RT1 class Ia, locus A1 [RT1-A1], and MHC class I RT1.Aa alpha-chain [Rt1.aa]), six were related to signal transduction (Olfactory receptor 1250 [Olr1250], Olfactory receptor 1315 [Olr1315], 24-dehydrocholesterol reductase [Dhcr24], Olfactory receptor 675 [Olr1250], Olfactory receptor 53 [Olr53], and Mitogen-activated protein kinase 8 interacting protein 2 [Mapk8ip2]), and three were related to cell proliferation (Protein phosphatase 2 [formerly 2A], regulatory subunit B [PR 52], beta isoform [Ppp2r2b], Interleukin 25 [Il25], and Met proto-oncogene [Met]). Similar to SMP3 mannosyltransferase (LOC684506) was related to protein biosynthesis, while Epithelial cell transforming sequence 2 oncogene (Ect2) was related to apoptosis.
In microarray analysis, 487 genes showed more than two fold up-regulation of expression while 437 genes were down-regulated in the M treatment group compared with the U treatment group (Table 1). The expressed genes which had a 7-fold increase are summarized in Table 4. Five genes (RT1 class II, locus Bb [RT1-Bb], Similar to ribosomal protein L6 [LOC685106], Intelectin 1 [galactofuranose binding] [Itln1], CART prepropeptide [Cartpt], and Structural maintenance of chromosomes 2 [Smc2]) were related to transport, six were related to signal transduction (THAP domain containing, Apoptosis associated protein 1 [Thap1], Olfactory receptor 157 [Olr157], Forkhead box N4 [Foxe3], Chemokine [C-C motif] ligand 20 [Ccl20], Interferon gamma [Ifng], and Paired immunoglobin-like type 2 receptor alpha [Pilra]), four were related to immune response (Olfactory receptor 413 [Olr413], Forkhead box E3 [Foxe3], Dopamine receptor D2 [Drd2], and Regenerating islet-derived 3 beta [Reg3b]), and three were related to cell differentiation (Glial fibrillary acidic protein [Gfap], Nuclear transport factor 2-like export factor 2 [Nxt2], and Outer dense fiber of sperm tails 1 [Odf1]). Estrogen receptor 1 [Esr1] was related to protein biosynthesis, and 5-Hydroxytryptamine (serotonin) receptor 7 (Htr7) was related to apoptosis. The genes that showed decreased expressions are summarized in Table 5. Four genes (Cannabinoid receptor 1 [Cnr1], Tocopherol [alpha] transfer protein [Ttpa], Solute carrier family 4, sodium bicarbonate cotransporter, member 5 [Slc4a5], and Tubulin, alpha 3B [Tuba3b]) were related to transport, five were related to signal transduction (AT rich interactive domain 5B [Arid5b], Olfactory receptor 1583 [Olr1583], MCF.2 cell line derived transforming sequence-like [Mcf21], Lectin, galactoside-binding, soluble, 9 [Lgals9], and RAB44, member RAS oncogene family [Rab44]), and two were related to stress response (Myeloperoxidase [Mpo], and Transmembrane protease, serine 6 [Tmpress6]). Tumor necrosis factor receptor superfamily, member 14 [Tnfrsf14] was related to cell proliferation, Protein disulfide isomerase family A, member 2 [Pdia2] was related to inflammatory response, and Cytochrome P450, family 2, subfamily d, polypeptide 4 [Cyp2d4v1] was related to lipid metabolism. Three genes (Ring finger protein 2 [Rnf2], Transcription factor 21 [Tcf21], and Zinc finger, CCHC domain containing 12 [Zcchc12]) were related to transcription, three were related to apoptosis (Apolipoprotein E [Apoe], Enhancer of polycomb homolog 1 [Epc1], and Transcription factor Dp-2 [Tfdp2]), and two were related to immune response (Killer cell lectin-like receptor, subfamily A, member 5 [Klra5], and ATP-binding cassette, sub-family A [ABC1], member 1 [Abca1]).
Human UCB-MSCs were found to grow uniformly. The cells were positive for the surface markers CD73 and CD105, but negative for the hematopoietic cell-specific surface markers CD14 and CD34. The gray lines in Fig. 1 indicate the isotype matched the mouse Ig G antibody control labeling. Human UCB-MSCs were able to be identified at passage 5 with CD73+, CD105+. Human MSCs do not express hematopoietic markers, CD73-, CD105-. Characterization of human UCB-MSCs by flow cytometry was performed with cultured cells.
The genes that showed differential expression by more than 2.0-fold (P<0.05) in at least one sample are shown in Figs. 2 and 3. The red color indicates over expression, while green indicates under expression (Fig. 4). Through microarray analysis, changes in the expression of genes associated with inflammatory response, immune response, lipid metabolism, cell adhesion, cell migration, cell differentiation, apoptosis, cell growth, transport, cell proliferation, transcription, and signal transduction were observed.
In this study, it was demonstrated that human UCB-MSCs (3×106/ml/cm2) which were injected via the external jugular vein were engrafted in the lung tissues of MCT-induced PAH rats. Adverse effects were not observed after the transfusion of human UCB-MSCs. We have demonstrated changes of gene expressions after bone marrow cell transfusion in MCT model of PAH in our previous study [27] and confirmed in the present study. Previously, Umar et al. [28], also reported the application of MSCs from donor rats with PAH reduces RV pressure overload, RV dysfunction, and lung pathology in recipient rats with PAH. Several studies have suggested that immune activation and the secretion of cytokines contribute to the pathogenesis of PAH [29, 30, 31, 32]. Although the etiology of PAH remains unknown despite extensive investigation, the incidence of PAH patients continues to increase in many countries [33, 34, 35, 36]. There is no doubt that human UCB-MSCs have therapeutic utility in the treatment of PAH. Infusion of high doses of human UCB-MSCs effectively reduces systemic inflammation and prevents the development of lung lesions in PAH. Several mechanisms may explain the antiinflammatory effects of human UCB-MSCs in this disease [37, 38, 39]. To investigate the mechanisms underlying the therapeutic effects of human UCB-MSCs, the gene expression profiles of lung tissue obtained 4 weeks after the injection of human UCB-MSCs in PAH rats were examined. The advantage of DNA microarray is that it can simultaneously evaluate changes in the relative expression of thousands of genes [22, 23, 24]. To gain further insight into the mechanism of human UCB-MSCs related to immune processes and genetic factors, the difference of gene expression between the groups injected with human MSCs and UCB-MSCs, the M group and the U group, were investigated. In addition, the difference of gene expression levels after human UCB-MSCs therapy was compared to identify potential candidate genes that might link the systemic immune response to the development of PAH disease by examining the gene expression patterns between the M group and the U group in PAH rats. In the present study, many immunologic processes and genetic factors were attributed to the pathogenesis of PAH. Immunologic abnormalities in the M group of PAH rats reflected marked activation of the immune system, leading to increased cytokine production. The data indicated that there were several genes with differential expression in the U group compared to the M group. The change in expression levels of several genes were confirmed after the injection of human UCB-MSCs. These genes were related to inflammatory response, immune response, lipid metabolism, cell adhesion, cell migration, cell differentiation, apoptosis, cell growth, transport, cell proliferation, transcription, and signal transduction.
Previous reports suggested that naive or gene-modified endothelial progenitors from peripheral blood or bone marrow can ameliorate some of the symptoms of MCT-induced pulmonary hypertension [40, 41]. In the investigation of PAH, gene microarrays have been employed in a variety of study designs performed on a diverse array of cell types and animal species. Animal microarray studies have been performed on both whole lung tissue and microdissected pulmonary vasculature of hypoxic and MCT-induced PAH [42, 43]. These studies have employed "hypothesis-building" strategies, helping to focus attention on potentially novel pathologic pathways. However, gene expression has also been utilized as a biomarker, potentially useful in the classification or identification of an individual's risk of disease [44]. Examination of gene expression profiling from the lungs of two genetically engineered mouse models provided important clues into the pathogenesis of transforming growth factor α (TGF-α)-induced pulmonary fibrosis and disruption of the BMPR2 pathway. The work by Hardie et al. [45]. utilized an advanced tetracycline-inducible transgenic induction of TGF-α with lung specificity.
To define of PAH genes, the scleroderma group was subcategorized into mild and severe. Genes that appeared to correlate with severity of disease included ILA, vascular endothelial growth factor, interleukin-β, and matrix metalloproteinase 9. Moreover, confirmation of previously differentially expressed genes by the study of Bull et al. [22] included ADM, IL7R, ZFP36, GLUL, JUND, and BCL6. To create effective and reliable cell-based therapies for pulmonary hypertension, it is important to clearly identify the population or subpopulations of nonhematopoietic stem or progenitor cells that contribute to particular tissues that provide large numbers of reparative cells while maintaining their differentiation capacity and ability to durably engraft in vivo [46]. Despite the injection of human UCB-MSCs into MCT-induced PAH rats, relatively little is yet known about the relationship between the pulmonary hypertension and MSCs. Therefore, in the current study, we was to investigate the changes of gene expression by microarray analysis after the injection of human UCB-MSCs into MCT-induced PAH rats. Many studies estimated that many of the patients do not respond to a single injection of human UCB-MSCs, and the risk of aneurysm formation is higher in the unresponsive group than among rats who defervesce completely after a single injection of human UCB-MSCs. The limitations of the present study were as follows. The sample size was small, and further analysis with larger samples of other independent sets as well as specific samples such as peripheral blood T cells, monocytes/macrophages would be needed to confirm the results of human UCB-MSCs injection.
Figures and Tables
Fig. 1
Human umbilical cord blood (UCB)-mesenchymal stem cell (MSCs) preparation. Characterization of human UCB-MSCs at passage 5 (A-D). Immunophenotype from human UCB-MSCs. These cells positive for antigens CD73 and CD105 but generally not for antigens CD14 and CD34 but generally not for. The gray lines indicate the isotype matched mouse IgG antibody control labeling (A, B, negative for the surface markers; C, D, positive for the surface markers.).

Fig. 2
The intensity plot demonstrate the consistency and correction of these array experiments (A-C). The expression profiles from the M group and U group were compared with those of the reference C group. The genes in which the detected signal showed a more than 2-fold difference between the samples fall outside of thin lines near the center of the scatterplot, respectively. C, control group; M, monocrotaline group; U, human umbilical cord blood-mesenchymal stem cells group.
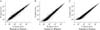
Fig. 3
The M versus A plot. M versus A plot showing genes identified as differentially expressed (2-fold or more, P≤0.05) between U, M, and C group between the samples fall outside of thin lines near the center of the scatterplot, respectively (A, B). C, control group; M, monocrotaline group; U, human umbilical cord blood-mesenchymal stem cells group.

Fig. 4
Gene heat map in pulmonary arterial hypertension (PAH) rats and human umbilical cord blood (UCB)-mesenchymal stem cells (MSCs) injection. The expression profiles of heat shock-activated genes were clustered hierarchically and are displayed using a red-green heat map. Heat map of genes upregulated and down-regulated ≥2 fold in PAH rats and human UCB-MSCs injection. The color red indicates over expression while green indicates down expression. C, control group; M, monocrotaline group; U, human UCB-MSCs group.
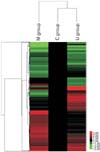
Acknowledgements
This research was supported by Basic Science Research Program through the National Research Foundation of Korea (NRF) funded by the Ministry of Education (2013R1A1A3004619).
References
1. Paffett ML, Hesterman J, Candelaria G, Lucas S, Anderson T, Irwin D, Hoppin J, Norenberg J, Campen MJ. Longitudinal in vivo SPECT/CT imaging reveals morphological changes and cardiopulmonary apoptosis in a rodent model of pulmonary arterial hypertension. PLoS One. 2012; 7:e40910.
2. Liang OD, Mitsialis SA, Chang MS, Vergadi E, Lee C, Aslam M, Fernandez-Gonzalez A, Liu X, Baveja R, Kourembanas S. Mesenchymal stromal cells expressing heme oxygenase-1 reverse pulmonary hypertension. Stem Cells. 2011; 29:99–107.
3. Fukumoto Y, Shimokawa H. Recent progress in the management of pulmonary hypertension. Circ J. 2011; 75:1801–1810.
4. Li B, Yang L, Shen J, Wang C, Jiang Z. The antiproliferative effect of sildenafil on pulmonary artery smooth muscle cells is mediated via upregulation of mitogen-activated protein kinase phosphatase-1 and degradation of extracellular signal-regulated kinase 1/2 phosphorylation. Anesth Analg. 2007; 105:1034–1041.
5. Can MM, TanboğZa IH, Demircan HC, Ozkan A, Koca F, Keleş N, Sönmez K, Kaymaz C, Serebruany V. Enhanced hemostatic indices in patients with pulmonary arterial hypertension: an observational study. Thromb Res. 2010; 126:280–282.
6. Zhao YD, Courtman DW, Ng DS, Robb MJ, Deng YP, Trogadis J, Han RN, Stewart DJ. Microvascular regeneration in established pulmonary hypertension by angiogenic gene transfer. Am J Respir Cell Mol Biol. 2006; 35:182–189.
7. Jiang Y, Jahagirdar BN, Reinhardt RL, Schwartz RE, Keene CD, Ortiz-Gonzalez XR, Reyes M, Lenvik T, Lund T, Blackstad M, Du J, Aldrich S, Lisberg A, Low WC, Largaespada DA, Verfaillie CM. Pluripotency of mesenchymal stem cells derived from adult marrow. Nature. 2002; 418:41–49.
8. Petersen BE, Bowen WC, Patrene KD, Mars WM, Sullivan AK, Murase N, Boggs SS, Greenberger JS, Goff JP. Bone marrow as a potential source of hepatic oval cells. Science. 1999; 284:1168–1170.
9. Prockop DJ. Marrow stromal cells as stem cells for non-hematopoietic tissues. Science. 1997; 276:71–74.
10. Prockop DJ, Gregory CA, Spees JL. One strategy for cell and gene therapy: harnessing the power of adult stem cells to repair tissues. Proc Natl Acad Sci U S A. 2003; 100:Suppl 1. 11917–11923.
11. Schwartz RE, Reyes M, Koodie L, Jiang Y, Blackstad M, Lund T, Lenvik T, Johnson S, Hu WS, Verfaillie CM. Multipotent adult progenitor cells from bone marrow differentiate into functional hepatocyte-like cells. J Clin Invest. 2002; 109:1291–1302.
12. Friedenstein AJ, Piatetzky-Shapiro II, Petrakova KV. Osteogenesis in transplants of bone marrow cells. J Embryol Exp Morphol. 1966; 16:381–390.
13. Pittenger MF, Mackay AM, Beck SC, Jaiswal RK, Douglas R, Mosca JD, Moorman MA, Simonetti DW, Craig S, Marshak DR. Multilineage potential of adult human mesenchymal stem cells. Science. 1999; 284:143–147.
14. Zuk PA, Zhu M, Mizuno H, Huang J, Futrell JW, Katz AJ, Benhaim P, Lorenz HP, Hedrick MH. Multilineage cells from human adipose tissue: implications for cell-based therapies. Tissue Eng. 2001; 7:211–228.
15. Bieback K, Kern S, Klüter H, Eichler H. Critical parameters for the isolation of mesenchymal stem cells from umbilical cord blood. Stem Cells. 2004; 22:625–634.
16. Erices A, Conget P, Minguell JJ. Mesenchymal progenitor cells in human umbilical cord blood. Br J Haematol. 2000; 109:235–242.
17. Goodwin HS, Bicknese AR, Chien SN, Bogucki BD, Quinn CO, Wall DA. Multilineage differentiation activity by cells isolated from umbilical cord blood: expression of bone, fat, and neural markers. Biol Blood Marrow Transplant. 2001; 7:581–588.
18. Kogler G, Sensken S, Airey JA, Trapp T, Müschen M, Feldhahn N, Liedtke S, Sorg RV, Fischer J, Rosenbaum C, Greschat S, Knipper A, Bender J, Degistirici O, Gao J, Caplan AI, Colletti EJ, Almeida-Porada G, Müller HW, Zanjani E, Wernet P. A new human somatic stem cell from placental cord blood with intrinsic pluripotent differentiation potential. J Exp Med. 2004; 200:123–135.
19. Zvaifler NJ, Marinova-Mutafchieva L, Adams G, Edwards CJ, Moss J, Burger JA, Maini RN. Mesenchymal precursor cells in the blood of normal individuals. Arthritis Res. 2000; 2:477–488.
20. Kuznetsov SA, Mankani MH, Gronthos S, Satomura K, Bianco P, Robey PG. Circulating skeletal stem cells. J Cell Biol. 2001; 153:1133–1140.
21. Javazon EH, Beggs KJ, Flake AW. Mesenchymal stem cells: paradoxes of passaging. Exp Hematol. 2004; 32:414–425.
22. Bull TM, Coldren CD, Moore M, Sotto-Santiago SM, Pham DV, Nana-Sinkam SP, Voelkel NF, Geraci MW. Gene microarray analysis of peripheral blood cells in pulmonary arterial hypertension. Am J Respir Crit Care Med. 2004; 170:911–919.
23. Menon S, Fessel J, West J. Microarray studies in pulmonary arterial hypertension. Int J Clin Pract Suppl. 2011; (169):19–28.
24. Rajkumar R, Konishi K, Richards TJ, Ishizawar DC, Wiechert AC, Kaminski N, Ahmad F. Genomewide RNA expression profiling in lung identifies distinct signatures in idiopathic pulmonary arterial hypertension and secondary pulmonary hypertension. Am J Physiol Heart Circ Physiol. 2010; 298:H1235–H1248.
25. Lee JC, Choe SY, Cha CI. Region-specific changes in the immunoreactivity of Atg9A in the central nervous system of SOD1(G93A) transgenic mice. Anat Cell Biol. 2014; 47:101–110.
26. Kim ES, Chang YS, Choi SJ, Kim JK, Yoo HS, Ahn SY, Sung DK, Kim SY, Park YR, Park WS. Intratracheal transplantation of human umbilical cord blood-derived mesenchymal stem cells attenuates Escherichia coli-induced acute lung injury in mice. Respir Res. 2011; 12:108.
27. Kim KC, Lee HR, Kim SJ, Cho MS, Hong YM. Changes of gene expression after bone marrow cell transfusion in rats with monocrotaline-induced pulmonary hypertension. J Korean Med Sci. 2012; 27:605–613.
28. Umar S, de Visser YP, Steendijk P, Schutte CI, Laghmani el H, Wagenaar GT, Bax WH, Mantikou E, Pijnappels DA, Atsma DE, Schalij MJ, van der Wall EE, van der Laarse A. Allogenic stem cell therapy improves right ventricular function by improving lung pathology in rats with pulmonary hypertension. Am J Physiol Heart Circ Physiol. 2009; 297:H1606–H1616.
29. Daley E, Emson C, Guignabert C, de Waal Malefyt R, Louten J, Kurup VP, Hogaboam C, Taraseviciene-Stewart L, Voelkel NF, Rabinovitch M, Grunig E, Grunig G. Pulmonary arterial remodeling induced by a Th2 immune response. J Exp Med. 2008; 205:361–372.
30. El Chami H, Hassoun PM. Immune and inflammatory mechanisms in pulmonary arterial hypertension. Prog Cardiovasc Dis. 2012; 55:218–228.
31. George PM, Badiger R, Shao D, Edwards MR, Wort SJ, Paul-Clark MJ, Mitchell JA. Viral Toll like receptor activation of pulmonary vascular smooth muscle cells results in endothelin-1 generation; relevance to pathogenesis of pulmonary arterial hypertension. Biochem Biophys Res Commun. 2012; 426:486–491.
32. Ross DJ, Strieter RM, Fishbein MC, Ardehali A, Belperio JA. Type I immune response cytokine-chemokine cascade is associated with pulmonary arterial hypertension. J Heart Lung Transplant. 2012; 31:865–873.
33. Aschermann M, Jansa P. Drug therapy of pulmonary arterial hypertension in 2014. Vnitr Lek. 2014; 60:282–288.
34. Idrees MM, Swiston J, Nizami I, Al Dalaan A, Levy RD. Saudi Guidelines on the Diagnosis and Treatment of Pulmonary Hypertension: medical and surgical management for pulmonary arterial hypertension. Ann Thorac Med. 2014; 9:Suppl 1. S79–S91.
35. Raskin J, Qua D, Marks T, Sulica R. A retrospective study on the effects of pulmonary rehabilitation in patients with pulmonary hypertension. Chron Respir Dis. 2014; 11:153–162.
36. Seferian A, Simonneau G. Pulmonary hypertension: definition, diagnostic and new classification. Presse Med. 2014; 43:935–944.
37. Han DM, Wang ZD, Ding L, Zheng XL, Yan HM, Xue M, Zhu L, Liu J, Wang HX. Effect of umbilical cord MSC infusion on the pulmonary infection in haploidentical hematopietic stem cell transplantation. Zhongguo Shi Yan Xue Ye Xue Za Zhi. 2014; 22:1084–1088.
38. Lee C, Mitsialis SA, Aslam M, Vitali SH, Vergadi E, Konstantinou G, Sdrimas K, Fernandez-Gonzalez A, Kourembanas S. Exosomes mediate the cytoprotective action of mesenchymal stromal cells on hypoxia-induced pulmonary hypertension. Circulation. 2012; 126:2601–2611.
39. Wang HY, Liu C, Wang Y, Zhang LL, Liu XR, Liu HL. Experimental treatment of pulmonary interstitial fibrosis with human umbilical cord blood mesenchymal stem cells. Zhonghua Lao Dong Wei Sheng Zhi Ye Bing Za Zhi. 2013; 31:675–680.
40. Nagaya N, Kangawa K, Kanda M, Uematsu M, Horio T, Fukuyama N, Hino J, Harada-Shiba M, Okumura H, Tabata Y, Mochizuki N, Chiba Y, Nishioka K, Miyatake K, Asahara T, Hara H, Mori H. Hybrid cell-gene therapy for pulmonary hypertension based on phagocytosing action of endothelial progenitor cells. Circulation. 2003; 108:889–895.
41. Zhao YD, Courtman DW, Deng Y, Kugathasan L, Zhang Q, Stewart DJ. Rescue of monocrotaline-induced pulmonary arterial hypertension using bone marrow-derived endotheliallike progenitor cells: efficacy of combined cell and eNOS gene therapy in established disease. Circ Res. 2005; 96:442–450.
42. Henke-Gendo C, Mengel M, Hoeper MM, Alkharsah K, Schulz TF. Absence of Kaposi's sarcoma-associated herpesvirus in patients with pulmonary arterial hypertension. Am J Respir Crit Care Med. 2005; 172:1581–1585.
43. Hoshikawa Y, Nana-Sinkam P, Moore MD, Sotto-Santiago S, Phang T, Keith RL, Morris KG, Kondo T, Tuder RM, Voelkel NF, Geraci MW. Hypoxia induces different genes in the lungs of rats compared with mice. Physiol Genomics. 2003; 12:209–219.
44. Buermans HP, Redout EM, Schiel AE, Musters RJ, Zuidwijk M, Eijk PP, van Hardeveld C, Kasanmoentalib S, Visser FC, Ylstra B, Simonides WS. Microarray analysis reveals pivotal divergent mRNA expression profiles early in the development of either compensated ventricular hypertrophy or heart failure. Physiol Genomics. 2005; 21:314–323.
45. Hardie WD, Korfhagen TR, Sartor MA, Prestridge A, Medvedovic M, Le Cras TD, Ikegami M, Wesselkamper SC, Davidson C, Dietsch M, Nichols W, Whitsett JA, Leikauf GD. Genomic profile of matrix and vasculature remodeling in TGFalpha induced pulmonary fibrosis. Am J Respir Cell Mol Biol. 2007; 37:309–321.
46. Spees JL, Whitney MJ, Sullivan DE, Lasky JA, Laboy M, Ylostalo J, Prockop DJ. Bone marrow progenitor cells contribute to repair and remodeling of the lung and heart in a rat model of progressive pulmonary hypertension. FASEB J. 2008; 22:1226–1236.