Abstract
Phlorotannins (marine algal polyphenols) have been reported to exhibit beneficial biological activities, serving as both antioxidants and anti-inflammatory agents. Among marine algae, Ecklonia cava, a member of the Laminariaceae, is a very popular food regarded as healthy in Korea and Japan. Recently, benefits afforded by phlorotannins in the treatment of various clinical conditions have been reported, but any therapeutic effects of such materials in the treatment of neurodegenerative diseases such as stroke remain unclear. Also, the mechanisms of action of the algal components remain poorly understood. In the present in vivo study, administration of Ecklonia cava polyphenols (ECP) at 10 mg/kg and 50 mg/kg intraperitoneally (i.p.) significantly decreased infarct size and the extent of brain edema in the rat after induction of transient focal ischemia via middle cerebral artery occlusion (MCAO). Further, terminal deoxynucleotidyl transferase-mediated dUTP nick end-labeling (TUNEL) assay revealed dose-dependent blockage of neuronal apoptosis upon intravenous ECP treatment. Neurobehavioral tests performed over the 6 days after MCAO revealed a reduction in neurological motor performance in control animals, but administration of ECP (50 mg/kg i.p.) prevented this decline. In vitro, a significant neuroprotective effect of ECP was evident when cell viability was assayed after induction of H2O2-mediated oxidative stress, upon retinoic acid treatment, in the differentiated neuroblastoma cell line SH-SY5Y. Interestingly, ECP blocked the rise in cytosolic calcium, in a dose-dependent manner, in differentiated SH-SY5Y cells exposed to H2O2. Together, the results suggest that ECP exerts neuroprotective effects in the focally ischemic brain by reducing Ca2+-mediated neurotoxicity.
Several studies on the cytotoxic action of Ca2+ ions have appeared over the last 30 years [1-3]. In the nervous system, Ca2+ overload can trigger various downstream cytotoxic cascades, including the uncoupling of electron transfer from adenosine triphosphate (ATP) synthesis in mitochondria, and activation of proteases, protein kinases, nitric oxide synthase, and endonucleases [4]. Such alterations in enzymatic activities can result in overproduction of reactive oxygen species (ROS) including nitric oxide (NO), superoxide (O2-), and hydrogen peroxide (H2O2). Further, these changes can induce failure of cytoskeletal organization, activation of apoptotic signals leading to cell death, and mitochondrial dysfunction [5]. In turn, adversely, the ROS-induced cytotoxic downstream cascades may render Ca2+ homeostasis dysfunctional and generate Ca2+ overload; the positive feedback loop thus created eventually kills the cell. Therefore, it is apparent that synthetic drugs and compounds of natural origin that diminish ROS levels per se or that activate enzymes with antioxidant properties may inhibit the rise in intracellular calcium ([Ca2+]i), thus preventing apoptotic neuronal death.
The beneficial effects of polyphenols (which have powerful antioxidant actions) have been thoroughly explored. Such compounds are favorite candidates for prevention of catastrophic neuronal events. Recent reports have greatly added to the store of relevant information. Polyphenols are natural com pounds with a range of phenolic structures that are present at high levels in vegetables, fruits, grains, roots, flowers, seeds, and teas [6]. Polyphenols are known to possess potent antioxidant properties; most studies on the health benefits afforded by these compounds have focused on the treatment of vascular diseases and cancers [7-9]. Polyphenols have been implicated in the prevention of neurodegenerative diseases, including stroke. The compounds protect neurons against oxidative damage and attenuate ischemic injury by interfering with the activity of inducible nitric oxide synthase, thus inhibiting lipid peroxidation and decreasing the extent of glial activation during reperfusion after a prior ischemic insult. This reduces the extent of the neuroinflammatory response [10]. Epidemiological studies have shown that high-level consumption of polyphenolic compounds can lower the risk of stroke [11, 12]. In addition, an increasing number of reports have evaluated the antioxidant properties of polyphenols from diverse natural products, focusing on possible neuroprotective actions. In this context, only very limited information is available on the polyphenols of marine plants. Among the natural sources of polyphenolic com pounds, brown algae is very popular as a health food in Korea and Japan. Ecklonia cava, a representative perennial brown alga of the Ecklonia group, is a member of the Laminariaceae family of the order Laminariales [13]. Marine algal polyphenols, termed phlorotannins, known from only brown algae, have been reported to exhibit several biological activities, including an antioxidative capacity [14, 15], an antidiabetic action [16], and an anti-amnesia ability [17]. However, the protective effects of phlorotannins from Ecklonia cava in terms of the development of cerebral ischemia have only rarely been investigated. Our earlier study revealed that a polyphenol-containing Ecklonia cava extract exerted a neuroprotective effect in animal models of global ischemia (data not shown; manuscript submitted).
In the present study, we investigated the efficacy of Ecklonia cava polyphenols (ECP) in terms of protection against middle cerebral artery occlusion (MCAO)-induced focal cerebral ischemia in rats. We measured infarct volume and the extent of brain edema. To evaluate the protective effects of ECP in terms of neuronal apoptosis of the damaged cerebral cortex and striatum, and the neurological deficits caused by MCAO, we used the terminal deoxynucleotidyl transferase-mediated dUTP nick end-labeling (TUNEL) assay and neurological scoring to evaluate brain damage, employing the scaling system of Garcia et al. [18]. In vitro, we assayed the viability of a differentiated human neuroblastoma cell line, SH-SY5Y, after exposure to H2O2-induced oxidative stress, with or without ECP preconditioning. Finally, we performed Fura-2AM-loaded [Ca2+]i measurements to evaluate ECP-induced inhibition of [Ca2+]i-induced neurotoxicity.
A polyphenol extract from Ecklonia cava (ECP; Seapolynol™) was kindly supplied by Botamedi Inc. (Jeju, Korea). The total polyphenol content of ECP (the phloroglucinol equivalent) was 98.5%. Notable compounds in ECP, as identified by high-performance liquid chromatography (HPLC), were dieckol, 8,8'-bieckol, 6,6'-bieckol, and phlorofurofucoeckol A. We used a Waters CAPCELL PAK ODS column (4.6×250 mm) to conduct HPLC; the eluent was 30% (v/v) aqueous MeOH at a flow rate of 0.8 ml/min.
Male Sprague-Dawley (SD) rats (Rattus norvegicus) were obtained from the Experimental Animal Center, Konyang University, Daejeon, South Korea. Rats were used at 4 months of age, at a body weight of 300-350 g. The animals were housed in a conventional manner at 23℃ and 60% relative humidity with a 12-hour light/12-hour dark cycle, and had free access to water and food. Animal handling and care conformed to our institutional guidelines; these, in turn, comply with international law and policy (see the NIH Guide for the Care and Use of Laboratory Animals; NIH Publication No. 85-23, 1985, revised 1996). All experiments were designed to minimize the number of animals used and the suffering caused by our procedures.
A total of 64 adult male SD rats were used. The rats were divided into four groups, each of 16 individuals; the groups were termed sham-operated, operated, 10 mg/kg ECP injected, and 50 mg/kg ECP-injected. All injections were intraperitoneal. In the latter two experimental groups, half of the final dose of ECP (diluted in phosphate buffer) was given 30 minutes before, and the other half 30 minutes after surgery. The following surgical method was used. The right middle cerebral artery (MCA) was occluded for 90 minutes using a modification of the intraluminal-suture insertion method [19, 20], in which a nylon filament is introduced into the MCA. Employing this approach, the right common carotid artery was exposed and the occipital branches of the external carotid artery (ECA) were electrocauterized. A 4-0 monofilament nylon suture was next inserted, via the ECA, into the internal carotid artery and the MCA, located 20-22 mm from the carotid bifurcation. Prior to each procedure, the suture was coated with a poly-L-lysine solution to augment adhesion to the surrounding endothelium and to increase the reproducibility of the resulting infarct. The neck incision was next closed and each animal was allowed to waken from anesthesia. At 60 minutes after MCAO, each operated animal was tested, using a standardized neurobehavioral scale, to confirm the presence of a typical neurological deficit. Rats that did not show initial contralateral paralysis were excluded from further study. At 90 minutes after MCAO, rats were reanesthetized, the intraluminal suture was carefully removed, and each incision was closed. Rats in the sham-operated group were subjected to the same surgical procedure but the nylon suture was advanced for only about 10 mm and next immediately withdrawn. After surgery, all operated rats were observed carefully for signs of discomfort and each of the four groups was further divided into four subgroups (of four animals each). These subgroups were subjected to different experimental tests, as described below. For example, one randomly selected subgroup (n=4) from each initial group (n=16) was subjected to varying types of neurobehavioral tests over the next 6 days. The remaining subgroups (n=12 animals in total) were sacrificed at different times after ischemic reperfusion (four rats at each timepoint) after the conduct of the various experiments described below.
To determine infarct volume, rats of individual subgroups (n=4 in each subgroup) were decapitated 24 hours after initiation of the post-ischemic reperfusion that followed MCAO. Each brain was removed and sliced into coronal sections 2.0 mm in thickness. Five brain slices from each rat were incubated in 2% (w/v) TTC solution for 10 minutes at 37℃ and next transferred to 4% (v/v) paraformaldehyde solution for fixation. Normal brain tissue was uniformly red in color whereas infarcted regions were whitish. The first, third, and fifth post-fixation brain slices from each rat were photographed and the extent of the whitish areas calculated using the Adobe PhotoShop CS imaging system. To minimize artifacts in the infarct area produced by post-ischemic edema, and to correct for individual differences in brain volumes, the infarct volume was calculated as a percentage of the total brain area of each section. The images were not manipulated apart from adjustment of the background color to black.
To determine brain water content, rats in individual subgroups (n=4 for each subgroup) were decapitated 72 hours after commencement of the post-ischemic reperfusion that followed MCAO. Whole brains were obtained and the right hemispheres quickly isolated. Each sample was weighed on an electronic balance and dried in an oven at 100℃ for 24 hours [21]. Next, all samples were precisely weighed again and the water content (a percentage value) determined using the following formula: [(wet weight minus dry weight)/wet weight]×100%.
Rats in individual subgroups (n=4 in each subgroup) were anesthetized with sodium pentobarbital and transcardially perfused with 0.1 M phosphate-buffered saline, pH 7.4, followed by 4% (v/v) paraformaldehyde in 0.1 M phosphate buffer, pH 7.4. Brains were removed and post-fixed in the same material for 6 hours. Brain tissue was dehydrated by successive immersion (for 2 hours each time) in 50%, 70%, 80%, 90%, 95%, and 100% (all v/v) ethanol baths at room temperature. Next, the slices were successively immersed, each time for 1 hour, in fresh pure xylene and in molds containing melted paraffin (Sigma, St. Louis, MO, USA). For each brain, 5 µm-thick serial transverse sections of the region containing both the cerebral cortex and striatum were mounted on microscope slides. To detect neuronal apoptosis, TUNEL staining was performed according to the protocol of the DeadEnd™ TUNEL detection system (Santa Cruz Biochemistry, Santa Cruz, CA, USA). Briefly, for each rat, 10 randomly selected coronal paraffinized sections were deparaffinized in xylene, hydrated by immersion in baths featuring a (decreasing) ethanol gradient, and washed in distilled water. Next, each section was incubated with a nucleotide-labeling mixture and terminal deoxynucleotidyl transferase, for 1 hour at 37℃, to catalytically add the peroxidase-labeled digoxigenin nucleotide to DNA fragments. Nuclei displaying DNA cleavage were dark brown in appearance after addition of the chromogen 3,3'-diaminobenzidine tetrachloride (Sigma). We counted all TUNEL-positive cells in a single microscopic field of each of 10 randomly selected sections of each target area (the cerebral cortex and the striatum of the right hemisphere).
Neurological evaluation of rats of individual subgroups (n=4 in each subgroup) was performed by two observers blinded to treatment, using the neurological scoring system of Garcia et al. [18]. Briefly, on postoperative day 0 (PD 0; 2 hours after MCAO), PD 2, PD 4, and PD 6, animal behavior was evaluated employing six independent criteria. These were spontaneous activity, symmetry of movements, outstretching of the forelimbs, wall climbing, reaction to body touch, and response to touching of the vibrissae. The results of the first three tests were graded from 0 (severe deficit) to 3 (no deficit), but those of the latter three had a lowest score of 1. The grades were summed by each observer and the average scores obtained during two consecutive test sessions were calculated. The total score for each rat was 3 points at a minimum, and maximally 18.
SH-SY5Y human neuroblastoma cells were purchased from ATCC (Manassas, VA, USA). Cells were plated in Dulbecco's modification of Eagle's medium Nutrient Mixture : Ham's F-12 (1 : 1, v/v) medium supplemented with 10% (v/v) fetal bovine serum, penicillin (100 IU/ml), streptomycin (100 µg/ml), and L-glutamine (2 mM). The cells were grown on culture dishes precoated with poly-D-lysine (50 mg/ml in sterile water) overnight. Differentiation was induced by addition of 10 µM all-trans-retinoic acid (RA; Sigma) on day 2 after plating, and continued for 7 days. Every 48 hours, the medium was replaced with fresh medium containing 1% RA, supplemented with 1% (v/v) fetal bovine serum, 2 mM glutamine, and penicillin/streptomycin. Cultures were maintained at 37℃ in 95% air/5% CO2 (both v/v) in a humidified incubator. At 7 days after RA treatment, all cells exhibited extended neurite outgrowth. The RA-treated SH-SY5Y cells were used in the experiments described below.
SH-SY5Y cells were grown, in a differentiated state, at a density of 3×104 cells per well in poly-D-lysine-coated 96-well plates and maintained at 37℃ in a 5% (v/v) CO2 atmosphere. Cells of the experimental group were treated with varying amounts of ECP (0.01, 0.5, 1, 10, and 50 µg/ml) for 24 hours and further incubated after exposure to 10 mM H2O2 diluted in serum-free medium. Seven hours later, all cells were transferred to H2O2-free medium for 16 hours. Next, 50 µl amounts of MTT (from a 2 mg/ml stock solution; Sigma) were added and the cells incubated for an additional 4 hours. Dimethyl sulfoxide (DMSO; 150 µl) was added to each well to dissolve formazan crystals and 20 µl of Sorenson's glycine buffer was added. After 20 minutes of gentle shaking, the absorbance of each well was read at 570 nm using a microplate reader (BioTek Inst., Winooski, VT, USA).
SH-SY5Y cells were plated on coverslips for 2 days and further differentiated for 7 days by addition of 10 µM RA treatment. Each cell was incubated with ECP of varying amounts (0, 0.01, and 1 µg/ml) for 24 hours and then were loaded with 3 mM Fura-2AM (dissolved in DMSO), added to serum-free growth medium, at 37℃ for 1 hour in a CO2 incubator, and were next washed with HEPES-buffered physiological saline containing 2.5 mM glucose, 137 mM NaCl, 0.56 mM MgCl2, 4.7 mM KCl, 1 mM Na2HPO4, 10 mM HEPES (pH 7.4), and 1.28 mM CaCl2. The coverslips bearing Fura-2AM-labeled cells were mounted in a perfusion chamber (Warner Instruments, Hamden, CT, USA) containing HEPES-buffered physiological saline and examined by inverted fluorescent microscopy (Till Photonics, Munich, Germany). Samples were scanned every 1 second using excitation at 340 nm and measuring emission at 380 nm. After baseline [Ca2+]i values were obtained over 60 seconds, 10 mM H2O2 was added to the perfusion chamber and changes in [Ca2+]i were measured. All images were processed for analysis of any changes at the single-cell level. The results were expressed as relative fluorescence intensity values [22].
All data are presented as means±SEMs. Comparisons of the outcomes of different treatments were performed using Student's t-test (Sigma Plot ver. 8.02 software, SPSS Inc., Chicago, IL, USA). Differences with P<0.05 were considered to be statistically significant. Below, each "n" value refers to the number of separate experiments conducted.
In the present study, the neuroprotective effects of ECP in terms of focal ischemia were evaluated by measurement of infarcted areas and the extent of brain swelling. Twenty-four hours after reperfusion, infarcted areas were measured by TTC staining. Quantitative assessment of the total infarcted proportion of the brain areas of the first, third, and fifth sections showed that intraperitoneal injection of 50 mg/kg ECP significantly reduced infarction. However, the infarct area of the 10 mg/kg ECP group was not significantly reduced compared with that of the operated group (Fig. 1A, B). The mean infarct areas in three representative slices from the operated group and the 50 mg/kg ECP group were 37.35±3.75% and 19.52±5.25% of total brain area, respectively. The extent of brain edema was also significantly reduced by administration of 50 mg/kg ECP (Fig. 1C). Although the reduction in the extent of brain edema seen when ECP was given at 10 mg/kg was not statistically significant, a tendency toward dose-dependent reduction in the extent of such edema was evident after ECP treatment.
TUNEL-positive apoptotic neuronal cells were rarely present in either the cerebral cortex or the striatum of the sham-operated group (Fig. 2A). In contrast, the numbers of apoptotic neuronal cells in either area were significantly larger in the operated group. As expected, a significant dose-dependent attenuation in the extent of neuronal apoptosis in both regions was evident in the ECP-treated groups. When ECP was given at 50 mg/kg, the numbers of TUNEL-positive cells in 10 randomly selected sections from each area were significantly lower than those in the cerebral cortex (26.2±8.2 vs. 356.4±10.6, n=4, P<0.001) and striatum (30.6±18.2 vs. 255.8±20.4, n=4, P<0.001) of the operated group (Fig. 2B).
To determine the effect of ECP treatment on the development of ischemia-induced neurobehavioral deficits, postoperative motor deficits evident in animals treated with the two different doses of ECP (10 mg/kg and 50 mg/kg) were compared with those of the operated group (lacking ECP treatment) using the neurological scoring system of Garcia. Rats of the sham-operated group showed slight neurobehavioral deficits at PD 0, but all scores subsequently became normal. When the neurological scores of the group treated with 50 mg/kg ECP were compared with those of the operated group, a statistically significant enhancement of neurological performance was evident at all timepoints (12.5±1.6 vs. 9±1.8 at PD 2, 14.4±0.8 vs. 10.2±1.6 at PD 4, 14.8±1.8 vs. 11.4±1.6 at PD 6; n=4 for each comparison; P<0.05 vs. operated) except for PD 0 (Fig. 3).
To further understand the neuroprotective mechanisms of ECP, we explored whether ECP treatment protected differentiated SH-SY5Y neuronal cells from H2O2-induced oxidative stress. As shown in representative cell features, we regarded SH-SY5Y cells at DIV7 as RA-induced fully differentiated states and used it in further studies (Fig. 4A). Before conducting a cell viability assay using different doses of ECP in the context of H2O2-induced damage, we determined the maximal safe dose of ECP under such conditions (Fig. 4B). Additionally, we identified the timepoint at which cell viability was reduced to 50% of that of the control after exposure to 10 mM H2O2; this was 7 hours. (Fig. 4C). All experimental cells were transferred to normal growth medium at this time, allowed to recover for 17 hours, and the levels of MTT reduction recorded. Cells treated with ECP at concentrations of 0.1, 0.5, 1, or 10 µg/ml (dissolved in culture medium) for 24 hours prior to exposure to 10 mM H2O2 had viabilities of 60.18±5.24%, 65.35±7.36%, 68.72±3.14%, and 65.32±6.60% of that of control cells, respectively (P<0.05, P<0.01 vs. control) (Fig. 4D). Such a dose-dependent cytoprotective effect of ECP on differentiated neuroblastoma cells after challenge with H2O2-induced oxidative stress is in agreement with the results of the in vivo experiments.
As mentioned above, a rise of [Ca2+]i is induced by ROS-induced oxidative stress and is proposed to be a principal cause of neuronal death, attributable to ischemic conditioning, and we thus measured [Ca2+]i in differentiated SH-SY5Y cells after exposure to H2O2. As shown in Fig. 5, [Ca2+]i was rapidly increased by treatment with 10 mM H2O2. As a positive control, we incubated cells for 30 minutes with 30 µM verapamil, a non-selective calcium channel blocker, prior to exposure to 10 mM H2O2. As expected, this agent significantly inhibited the H2O2-induced elevation of [Ca2+]i. Similarly, 24 hours of ECP treatment (at 0.01 µg/ml or 1 µg/ml) prior to exposure to 10 mM H2O2 resulted in a dose-dependent inhibition of the H2O2-induced rise in [Ca2+]i. These results suggest that previously shown neuroprotective effects of ECP against oxidative stress could inhibit the elevation of [Ca2+]i and at least in part, may eventually increase neuronal survival.
Increasing evidence supports the hypothesis that polyphenols protect neurons against changes associated with cerebral ischemia [23]. Ischemic stroke is caused by an interruption of cerebral blood flow, which can lead to failure of energy production, activation of proteases, impairment of metabolism, free radical production, excitotoxicity, and altered calcium homeostasis [24-27]. Cerebral ischemia is associated with a severe deterioration in the ability of neuronal mitochondria to function effectively; this seriously compromises oxidative phosphorylation, a key mechanism by which ATP is produced. Apart from ATP depletion, dysfunctional mitochondria may increase ROS production and are unable to maintain a normal [Ca2+]i. This causes depolarization of the cytoplasmic membrane potential. Such changes can contribute to both Ca2+-induced membrane damage and increase levels of Ca2+-induced proteases, the extent of ROS-mediated cell damage (to membranes and DNA) and lipid peroxidation [28]. Thus, potential neuroprotectants seek to reduce oxidative stress and mitochondrial damage. It is thus reasonable to suggest that the therapeutic use of polyphenols (which possess strong antioxidant activities) may reduce cell damage, thus improving cell viability, following cerebral ischemia [29-31].
Recently, knowledge of the beneficial roles played by polyphenols derived from terrestrial plants in protection from neurodegenerative diseases such as stroke has greatly increased, but only very limited information is available on the polyphenol components of marine plants. Brown algae are popular health foods in Korea and Japan. Ecklonia cava, a representative perennial brown alga of the Ecklonia group, is a member of the Laminariaceae of the order Laminariales [13]. As mentioned above, purified polyphenols from Ecklonia cava have been reported to exert multiple biological activities, although their neuroprotective effect of ECP on stroke has received only limited attention. In our previous study, ECP protected hippocampal slices of the gerbil from global ischemia induced by bilateral common carotid artery occlusion, consistent with in vitro data showing that ECP protected against oxygen-glucose deprivation-induced cytotoxic death of the SH-SY5Y cell line. Although these results were the first experimental demonstration of the neuroprotective efficacy of ECP in the context of cerebral ischemia, some fundamental limitations of the work are evident.
First, global ischemia is not the only form of cerebral ischemia. It is generally accepted that two types of ischemia exist: these are global ischemia, affecting wide areas of brain tissue, and focal ischemia, confined to a specific region of the brain. The former condition occurs when blood flow to the brain is halted, or drastically reduced, as when cardiac arrest occurs. The latter condition eventuates when a blood clot occludes a cerebral vessel; indeed, the condition is clinically less common than focal ischemia. To overcome this limitation, in the present study we adapted the focal ischemia model to permit in vivo screening of the therapeutic efficacy of ECP in the context of cerebral ischemia.
Second, although the human neuroblastoma cell line, SH-SY5Y, is commonly employed for in vitro neurotoxicological testing, because the cells were originally derived from neurons, are very sensitive to neuronal inhibitors, and are morphologically similar to neurons, doubts have been expressed concerning functional mimicry of neurons by such cells. To overcome this limitation, in the present study we used RA-induced differentiated SH-SY5Y cells to conduct supportive in vitro experiments. Indeed, SH-SY5Y cells can differentiate, both morphologically and functionally, into neuron-like cells when cultured in the presence of low levels of RA [32-34]. Such differentiation, accompanied by neurite extension, is also associated with increased electrical excitability of the cytoplasmic membrane [35] and alterations in the expression levels of certain G-protein coupled receptor subunits [36]. Given that a neuron-like phenotype is shown by differentiated SH-SY5Y cells, the present study was undertaken to examine changes in cell viability and [Ca2+]i after pretreatment with different amounts of ECP.
We also considered the effects of ECP on post-ischemic brain edema. Such edema is an essential component of ischemic injury [37], being characterized by abnormal accumulation of exudate in the parenchyma, resulting in volumetric enlargement of the tissue. Acute brain edema usually radically affects clinical prognosis after occurrence of ischemia, because edema can lead to brain herniation, irreversible brain damage, and, eventually, death. Brain edema is of two distinct types, distinguished by the origin and location of fluid, and is classified as either cytotoxic or vasogenic [38]. The former is a neuronal form of swelling; fluid accumulates in the cytosol. Vasogenic edema is caused by breakdown of the blood-brain barrier, resulting in fluid accumulation in the extracellular space. Reductions in the levels of either type of edema may improve neurobehavioral morbidity [39] and recirculation in the cerebral microvasculature [40, 41]. As we have shown in the present study, ECP treatment can effectively reduce the extent of edema; this limits the extent of ischemia-induced secondary damage and may have a role to play in neuroprotection against ischemic injury [42].
In summary, we suggest that ECP plays a beneficial role in the treatment of cerebral ischemia and neurological deficits caused by this condition, at least partly by reducing the extent of brain edema, inhibiting neuronal apoptosis, and blocking the rise in cytotoxic [Ca2+]i. It remains to be established whether ECP is also helpful in the treatment of other neurodegenerative conditions including parkinsonism and Alzheimer's disease.
Figures and Tables
Fig. 1
Effects of Ecklonia cava polyphenols (ECP) on infarct area and the extent of brain edema in an in vivo rat model of focal cerebral ischemia. (A) Representative photographs showing that ECP (50 mg/kg) decreased the extent of infarction (infarcted areas appear whitish in color upon triphenyltetrazolium chloride staining). (B) Quantitation of the summed proportions of infarct area (in terms of total brain area) in the first, third, and fift h sections revealed that intraperitoneal injection of 50 mg/kg ECP significantly reduced the infarct area, compared to that of the operated group, whereas this was not the case when ECP was given at 10 mg/kg. The mean infarct areas in three representative slices from the operated group and the group treated with 50 mg/kg ECP were 37.35±3.75% and 19.52±5.25% of the total brain areas, respectively (n=4 per group, *P<0.05, significantly different from the operated group). (C) In ECP-treated groups, the brain water content was reduced, in a dose-dependent manner, compared to that of the operated group (n=4 per group, *P<0.05). The bars indicate means±SEMs. SO, sham-operated; OP, operated.
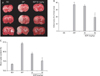
Fig. 2
Effects of Ecklonia cava polyphenols (ECP) on neuronal apop-tosis in the cerebral cortex and striatum of an in vivo model of focal cerebral ischemia. (A) Representative magnifications (×200) of terminal deoxynucleotidyl transferase-mediated dUTP nick end-labeling (TUNEL)-stained slices of the cerebral cortex (upper panel) and striatum (lower panel) revealed an anti-apoptotic dose-dependent effect of ECP in the context of focal cerebral ischemia. TUNEL-positive apoptotic neuronal cells were rarely seen in either the cerebral cortex or the striatum of the sham-operated group. In contrast, the numbers of apoptotic neuronal cells in either area were significantly increased in the operated group. A significant dose-dependent attenuation of neuronal apoptosis in either region was evident in the ECP-treated groups. Scale bar=500 µm. (B) TUNEL-immunopositive cell counts in 10 randomly selected sections of each area revealed that the total numbers of TUNEL-positive cells in the cerebral cortex (26.2±8.2 vs. 356.4±10.6, n=4 per group, ***P<0.001) and the striatum (30.6±18.2 vs. 255.8±20.4, n=4 per group, ***P<0.001) were significantly lower in rats treated with 50 mg/kg ECP compared to the operated group (**P<0.01). The bars indicate means±SEMs. SO, sham-operated; OP, operated.
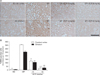
Fig. 3
Effects of Ecklonia cava polyphenols (ECP) on amelioration of neurological deficits evaluated using the neurological scoring system of Garcia. Rats of the sham-operated group showed slight neurobehavioral deficits at postoperative day (PD) 0, but all scores were normal at later timepoints. When the neurological scores of 50 mg/kg ECP-treated animals were compared with those of the operated group, a statistically significant enhancement of neurological performance was evident at all timepoints (12.5±1.6 vs. 9±1.8 at PD 2; 14.4±0.8 vs. 10.2±1.6 at PD 4; and 14.8±1.8 vs. 11.4±1.6 at PD 6; n=4 for each test; *P<0.05) except for that of PD 0. The bars show means±SEMs. SO, sham-operated; OP, operated; MCAO, middle cerebral artery occlusion.
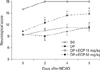
Fig. 4
Effect of Ecklonia cava polyphenols (ECP) on the viability of retinoic acid-induced differentiated SH-SY5Y cells after 7 days in vitro (DIV). (A) Representative microscopic images of naive SH-SY5Y cells at DIV 1 and differentiated states of cells at DIV 7 showing the morphologic differences between them such as abundant formation of neurite-like cellular processes. (B) To determine the maximal safe dose, differentiated SH-SY5Y cells were exposed to various concentrations of ECP (0.01-100 µg/ml) and cell viability was determined using the MTT assay (n=7 per group; *P<0.05, ***P<0.001). The results differed significantly from those from control cells (lacking any ECP treatment). (C) The time after exposure to 10 mM H2O2 at which cell viability was reduced to 50% of the control level was determined; this (7 h) was the optimal timepoint (indicated by the dotted line) for conduct of further cell viability studies (n=7 per group). (D) Cell viability assays were performed, using data from the experiments of Fig. 4B and C, and it was observed that cells treated with ECP at doses of 0.1, 0.5, 1, or 10 µg/ml for 24 h prior to exposure to 10 mM H2O2 showed increases in viability of 60.18±5.24%, 65.35±7.36%, 68.72±3.14%, and 65.32±6.60% that of the control, respectively (n=7 per group; *P<0.05, **P<0.01; significantly different from control). The bars show means±SEMs.
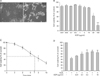
Fig. 5
Effect of Ecklonia cava polyphenols (ECP) on inhibition of the H2O2-induced rise in [Ca2+]i. The [Ca2+]i in differentiated SHSY5Y cells increased rapidly upon treatment with 10 mM H2O2. However, 30 min of incubation with 30 µM verapamil, a non-selective calcium channel blocker, prior to exposure to 10 mM H2O2, dramatically inhibited the H2O2-induced elevation of [Ca2+]i. Interestingly, 24 h of ECP treatment (at either 0.01 or 1 µg/ml) before exposure to 10 mM H2O2 resulted in a dose-dependent inhibition of the H2O2-induced rise in [Ca2+]i, similar to that seen using verapamil treatment as a positive control. Results are expressed as the relative fluorescence intensity (RFI). Each trace shows a single cell that is representative of four independent experiments.
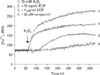
Acknowledgements
The authors would like to thank Miss Yeji Lee for technical assistance. The work was supported by a Korea Research Foundation Grant funded by the Korean Government (no. KRF-2012-0003314) and Konyang University Myunggok Research Fund of 2006.
References
1. Schlaepfer WW, Bunge RP. Effects of calcium ion concentration on the degeneration of amputated axons in tissue culture. J Cell Biol. 1973. 59(2 Pt 1):456–470.
2. Schanne FA, Kane AB, Young EE, Farber JL. Calcium dependence of toxic cell death: a final common pathway. Science. 1979. 206:700–702.
3. Choi DW. Glutamate neurotoxicity in cortical cell culture is calcium dependent. Neurosci Lett. 1985. 58:293–297.
4. Arundine M, Aarts M, Lau A, Tymianski M. Vulnerability of central neurons to secondary insults after in vitro mechanical stretch. J Neurosci. 2004. 24:8106–8123.
5. Szydlowska K, Tymianski M. Calcium, ischemia and excitotoxicity. Cell Calcium. 2010. 47:122–129.
6. Bravo L. Polyphenols: chemistry, dietary sources, metabolism, and nutritional significance. Nutr Rev. 1998. 56:317–333.
7. Rice-Evans CA, Miller NJ, Bolwell PG, Bramley PM, Pridham JB. The relative antioxidant activities of plant-derived polyphenolic flavonoids. Free Radic Res. 1995. 22:375–383.
8. Rice-Evans C, Miller N, Paganga G. Antioxidant properties of phenolic compounds. Trends Plant Sci. 1997. 2:152–159.
9. Sun AY, Chen YM. Oxidative stress and neurodegenerative disorders. J Biomed Sci. 1998. 5:401–414.
10. Nijveldt RJ, van Nood E, van Hoorn DE, Boelens PG, van Norren K, van Leeuwen PA. Flavonoids: a review of probable mechanisms of action and potential applications. Am J Clin Nutr. 2001. 74:418–425.
11. Acheson RM, Williams DR. Does consumption of fruit and vegetables protect against stroke? Lancet. 1983. 1:1191–1193.
12. Joshipura KJ, Ascherio A, Manson JE, Stampfer MJ, Rimm EB, Speizer FE, Hennekens CH, Spiegelman D, Willett WC. Fruit and vegetable intake in relation to risk of ischemic stroke. JAMA. 1999. 282:1233–1239.
13. Kang HS, Chung HY, Jung JH, Son BW, Choi JS. A new phlorotannin from the brown alga Ecklonia stolonifera. Chem Pharm Bull (Tokyo). 2003. 51:1012–1014.
14. Zou Y, Qian ZJ, Li Y, Kim MM, Lee SH, Kim SK. Antioxidant effects of phlorotannins isolated from Ishige okamurae in free radical mediated oxidative systems. J Agric Food Chem. 2008. 56:7001–7009.
15. Kang KA, Lee KH, Park JW, Lee NH, Na HK, Surh YJ, You HJ, Chung MH, Hyun JW. Triphlorethol-A induces heme oxygenase-1 via activation of ERK and NF-E2 related factor 2 transcription factor. FEBS Lett. 2007. 581:2000–2008.
16. Okada Y, Ishimaru A, Suzuki R, Okuyama T. A new phloroglucinol derivative from the brown alga Eisenia bicyclis: potential for the effective treatment of diabetic complications. J Nat Prod. 2004. 67:103–105.
17. Yoon NY, Chung HY, Kim HR, Choi JS. Acetyl- and butyrylcholinesterase inhibitory activities of sterols and phlorotannins from Ecklonia stolonifera. Fish Sci. 2008. 74:200–207.
18. Garcia JH, Wagner S, Liu KF, Hu XJ. Neurological deficit and extent of neuronal necrosis attributable to middle cerebral artery occlusion in rats. Statistical validation. Stroke. 1995. 26:627–634.
19. Belayev L, Alonso OF, Busto R, Zhao W, Ginsberg MD. Middle cerebral artery occlusion in the rat by intraluminal suture. Neurological and pathological evaluation of an improved model. Stroke. 1996. 27:1616–1622.
20. Longa EZ, Weinstein PR, Carlson S, Cummins R. Reversible middle cerebral artery occlusion without craniectomy in rats. Stroke. 1989. 20:84–91.
21. Schwab M, Bauer R, Zwiener U. The distribution of normal brain water content in Wistar rats and its increase due to ischemia. Brain Res. 1997. 749:82–87.
22. Nguyen TT, Cho SO, Ban JY, Kim JY, Ju HS, Koh SB, Song KS, Seong YH. Neuroprotective effect of Sanguisorbae Radix against oxidative stress-induced brain damage: in vitro and in vivo. Biol Pharm Bull. 2008. 31:2028–2035.
23. Simonyi A, Wang Q, Miller RL, Yusof M, Shelat PB, Sun AY, Sun GY. Polyphenols in cerebral ischemia: novel targets for neuroprotection. Mol Neurobiol. 2005. 31:135–147.
24. Wexler BC. Metabolic changes in response to acute cerebral ischemia following bilateral carotid artery ligation in arteriosclerotic versus nonarteriosclerotic rats. Stroke. 1970. 1:112–121.
25. Martins E, Inamura K, Themner K, Malmqvist KG, Siesjö BK. Accumulation of calcium and loss of potassium in the hippocampus following transient cerebral ischemia: a proton microprobe study. J Cereb Blood Flow Metab. 1988. 8:531–538.
26. Choi DW. Calcium-mediated neurotoxicity: relationship to specific channel types and role in ischemic damage. Trends Neurosci. 1988. 11:465–469.
27. Panickar KS, Norenberg MD. Astrocytes in cerebral ischemic injury: morphological and general considerations. Glia. 2005. 50:287–298.
28. Sims NR, Muyderman H. Mitochondria, oxidative metabolism and cell death in stroke. Biochim Biophys Acta. 2010. 1802:80–91.
29. Urquiaga I, Leighton F. Plant polyphenol antioxidants and oxidative stress. Biol Res. 2000. 33:55–64.
30. Dajas F, Rivera-Megret F, Blasina F, Arredondo F, Abin-Carriquiry JA, Costa G, Echeverry C, Lafon L, Heizen H, Ferreira M, Morquio A. Neuroprotection by flavonoids. Braz J Med Biol Res. 2003. 36:1613–1620.
31. Halliwell B. Are polyphenols antioxidants or pro-oxidants? What do we learn from cell culture and in vivo studies? Arch Biochem Biophys. 2008. 476:107–112.
32. Pahlman S, Ruusala AI, Abrahamsson L, Mattsson ME, Esscher T. Retinoic acid-induced differentiation of cultured human neuroblastoma cells: a comparison with phorbolester-induced differentiation. Cell Differ. 1984. 14:135–144.
33. Adem A, Mattsson ME, Nordberg A, Påhlman S. Muscarinic receptors in human SH-SY5Y neuroblastoma cell line: regulation by phorbol ester and retinoic acid-induced differentiation. Brain Res. 1987. 430:235–242.
34. Kaplan DR, Matsumoto K, Lucarelli E, Thiele CJ. Eukaryotic Signal Transduction Group. Induction of TrkB by retinoic acid mediates biologic responsiveness to BDNF and differentiation of human neuroblastoma cells. Neuron. 1993. 11:321–331.
35. Perez-Polo JR, Werbach-Perez K, Tiffany-Castiglioni E. A human clonal cell line model of differentiating neurons. Dev Biol. 1979. 71:341–355.
36. Ammer H, Schulz R. Retinoic acid-induced differentiation of human neuroblastoma SH-SY5Y cells is associated with changes in the abundance of G proteins. J Neurochem. 1994. 62:1310–1318.
37. Klatzo I. Presidental address. Neuropathological aspects of brain edema. J Neuropathol Exp Neurol. 1967. 26:1–14.
38. Katzman R, Clasen R, Klatzo I, Meyer JS, Pappius HM, Waltz AG. Report of Joint Committee for Stroke Resources. IV. Brain
edema in stroke. Stroke. 1977. 8:512–540.
39. Albers GW. Diffusion-weighted MRI for evaluation of acute stroke. Neurology. 1998. 51:3 Suppl 3. S47–S49.
40. Siesjö BK. Cerebral circulation and metabolism. J Neurosurg. 1984. 60:883–908.
41. Vexler ZS, Roberts TP, Bollen AW, Derugin N, Arieff AI. Transient cerebral ischemia. Association of apoptosis induction with hypoperfusion. J Clin Invest. 1997. 99:1453–1459.
42. Mellergård P, Bengtsson F, Smith ML, Riesenfeld V, Siesjö BK. Time course of early brain edema following reversible forebrain ischemia in rats. Stroke. 1989. 20:1565–1570.