Abstract
Corticotropin-releasing factor (CRF) is a peptide involved in the activation of the hypothalamic-pituitary-adrenal (HPA) axis. CRF is distributed not only along the HPA axis but also throughout pain-relevant anatomical sites. CRF elicits potent antinociception at the three main levels of pain transmissions: namely, the brain, spinal cord, and peripheral sensory neurons. The widespread distribution of CRF receptors 1 and 2 in the brain offers several targets wherein CRF could alter pain, some of which may be independent of the HPA axis. In this study, we assessed the expression of CRF and its receptors, CRF receptor type (CRFR)1 and CRFR2, in the spinal dorsal horn and dorsal root ganglion (DRG) in a rat model of neuropathic pain induced by spinal nerve injury (SNI). CRF was expressed in a few DRG neurons and primary afferent fibers in the dorsal horns of naїve rats, and the CRF-positive neurons in DRG and fibers in the spinal dorsal horn were found to have increased after SNI. CRFR1 was not expressed in DRG or the dorsal horn and CRFR2 was expressed weakly in the small neurons in DRG in the naїve rats. After SNI, CRFR1 was expressed in the activated microglia in the ipsilateral dorsal horn, and immunoreaction for CRFR2 was increased in the contralateral DRG following SNI. Consequently, it has been suggested that the increased expression of CRF and CRFR2 in DRG neurons and primary afferent fibers in dorsal horn, and CRFR1 in the activated microglia, may be involved in the mediation of stress responses as well as in microglial activation in the neuropathic pain state following SNI.
Corticotropin-releasing factor (CRF) is a peptide known to be involved in the activation of the hypothalamic-pituitary-adrenal (HPA) axis; CRF also performs a pivotal role in the body's responses to stress. The CRF family is composed of CRF and the CRF ligands mammalian, urocortin (Ucn) 1, and Ucn 2. The CRF receptor (CRFR) subtypes, CRFR1 and R2, evidence different affinity for CRF, Ucn1, Ucn 2, and Ucn 3 [1, 2].
CRF not only stimulates the pituitary adrenal axis but also influences a variety of central nervous system (CNS) functions [3]. CRF-positive neurons are located primarily within the paraventriculo-infundibular system and are also found in many areas of the CNS associated with the stress response, such as the amygdala and nucleus accumbens [4, 5]. CRF also functions as a neurotransmitter or neuromodulator in the sensory system. CRF-positive cells and fibers have previously been detected in the dorsal root ganglion (DRG) [6], lateral column, lamina I, V-VII, X, and in the intermediolateral column. The majority of CRF-positive fibers in spinal cords were believed to be ascending fibers, as they did not disappear after spinal cord transection [5]. The distribution of the CRF-immunoreactive fibers in the lumbosacral cord is similar to that of the pelvic nerve afferents; this raises the possibility that CRF may function as a transmitter in afferent neurons innvervating the pelvic viscera [7]. The receptors of CRF are known to be distributed broadly throughout the CNS, including in the spinal cord, as demonstrated by the results of earlier autoradiographic studies [8], In the spinal cord, CRFRs are highly concentrated in the superficial laminae [9] and also in lamina VII and lamina X.
The CRF is known to exert an antinociceptive effect, which is mediated by CRFRs in the HPA axis, the amygdala, and the spinal cord [9, 10]. The functional relation of CRF with pain is based on its expression in the anatomical sites of pain transmission as well as the HPA axis [11]. The local injection of CRF in the brain, spinal cord, and periphery has been shown to produce antinociceptive effects against inflammatory pain, and this action may be mediated by CRFRs in opiod-expressing interneurons [12]. The activation of endogenous CRFR1 in the amygdala also contributes to pain-related synaptic facilitation, increased excitability, and pain behavior [13, 14]. In previous immunohistochemical studies, CRFRs have been detected primarily in the areas of the spinal cord associated with visceral sensation [15-17]; however, the changes in CRF expression associated with the neuropathic pain state have yet to be clearly elucidated.
It has been suggested in recent studies that the immunological activity of the CRF is relevant to the peripheral antinociceptive effects of CRF [18], and also that CRF may regulate neuroinflammation by inducing microglial apoptosis [19]. Microglial activation after spinal nerve injury (SNI) is a major factor in the development of neuropathic pain [20].
We hypothesized that the expression of CRF and its receptors in the spinal cord and DRG neurons are altered in the neuropathic pain state, and that they may contribute to microglial activation. In this study, we evaluated changes in the expressions of CRF and its receptors CRFR1 and CRFR2 in the spinal cord and DRG in a rat model of peripheral nerve injury-induced neuropathic pain.
Twenty-five male Sprague-Dawley rats weighing 160-180 g each were used in this study. The rats were housed three per cage, and were provided with free access to water and food. All animal experiments were approved by the Institutional Animal Care and Use Committee of Hanyang University.
Neuropathic pain was induced via a modification of the spinal nerve ligation model [21]. The experimental animals were subdivided into naїve (5 rats), sham control (10 rats) and SNI groups (10 rats). The rats were anesthetized using a mixture of Zoletil (27.78 mg/kg, i.p.) and Xylazine (0.647 mg/kg, i.p.). The left transverse process of the L6 vertebra was removed through an incision on the skin of the back, and the L5 and L6 spinal nerves of the left side were transected and 1 mm segments of their distal ends were cut [22]. The sham control group operation was also conducted via the same procedure as mentioned above, with the exception of L5 and L6 SNI.
Neuropathic pain was evaluated by measuring the threshold of 50% paw withdrawal responses to mechanical stimulation. The rats were assessed for mechanical allodynia of the plantar surface of the hindpaw of the left side 2 days prior to surgery, and 1, 2, 4, 7, and 14 days after surgery by von Frey filaments. The rats were placed on the metal mesh floor under custom-made transparent acrylic cells without a floor and accommodated for 15 minutes. In an effort to assess mechanical allodynia, von Frey filaments (0.25, 0.65, 1.05, 1.56, 2.60, 4.89, 6.16, 8.40, 15.25, and 21.75 g) were pressed onto the plantar surface via an up-and-down paradigm beginning with the 6.16 g filament, and the 50% paw withdrawal response threshold was calculated as previously described [23].
Statistical analyses were conducted via one-way ANOVA using the SPSS ver. 12.0 (SPSS Inc., Chicago, IL, USA). The threshold for statistical significance was set at P<0.005.
The animals were perfused with 4% paraformaldehyde in phosphate buffer (PB, 0.1M, pH 7.4) at 7 and 14 days after surgery. The L5 DRG and spinal cord were fixed for 4 hours in the same solution and cryoprotected overnight in 30% sucrose in PB. Transverse sections of the L5 spinal cord (30 µm) and DRG (20 µm) were cut on a cryostat, blocked with 10% normal donkey serum (NDS; Jackson ImmunoResearch Laboratories, West Grove, PA, USA) in phosphate-buffered saline (PBS; 0.01M, pH 7.2) for 40 minutes, and incubated overnight with primary antibodies. For immunofluorescence, the sections were incubated with a mixture of the primary antibodies. The primary antibodies used were rabbit anti-CRF (1 : 1,000, Phoenix, Burlingame, CA, USA), goat anti-transient receptor potential vanilloid 1 receptor (TRPV1; 1 : 500, Neuromics, Edina, MN, USA), goat anti-CRFR1 (1 : 100, Santa Cruz, Santa Cruz, CA, USA), goat anti-CRFR2 (1 : 100, Santa Cruz) and rabbit anti-Iba1 (1 : 1,000, Wako, Osaka, Japan). The following day, the sections were rinsed and incubated for 15 minutes in 2% NDS and then incubated for 3 hours with a mixture of the secondary antibodies, anti-goat or anti-rabbit secondary antibodies conjugated to Cy3 (1 : 200, Jackson ImmunoResearch Laboratories) or Alexa 488 (1 : 200, Invitrogen, Carlsbad, CA, USA) in PBS. After washing, the sections were coverslipped with Vectashield (Vector Laboratories, Burlingame, CA, USA), and visualized on a Leica DMR microscope. Images were acquired with a CCD camera (Fluoview, SIS, Muenster, Germany) attached to the microscope or a confocal microscope (SP-5, Leica, Heidelberg, Germany).
To quantify the number of CRF-positive neurons in DRG, 15 DRG sections from 5 animals in each group were selected and the numbers of CRF-positive neurons were counted. To assess CRFR1 intensity in the spinal dorsal horn and CRFR2 intensity in the DRG, 15 spinal cord sections and DRG sections from 5 animals in each group were selected and the representative intensity was determined and expressed as follows: + (weak intensity), ++ (moderate intensity), +++ (strong intensity).
Mechanical hyperalgesia after SNI was evaluated via von Frey tests. In the SNI group, mechanical withdrawal threshold in the ipsilateral (left side) hindpaw was reduced significantly at 1 day after surgery compared to the control and sham animals, and the reduced levels were sustained until 14 days. In the sham group, the threshold was reduced at 1 day after surgery and recovered 2 days after surgery (Fig. 1).
Immunohistochemical CRF expression was assessed in the DRG and the spinal cord at the L5 level. TRPV1 immunohistochemistry was also carried out in the DRG to characterize the CRF positivity of the DRG neurons. In the DRG of naїve animals, strong immunoreactivity for CRF was noted in a few small cells; these cells were also stained for TRPV1. In ipsilateral DRG at the L5 level 7 and 14 days after SNI, virtually all CRF immunoreactivity disappeared. In the contralateral DRG of the SNI group, CRF-positive neurons were increased relative to the naїve and sham control groups (Fig. 2).
In the spinal cords of naїve rats, CRF immunoreactivity was observed in the fibers and puncta in lamina I-II of the dorsal horn. At 7 and 14 days after SNI, CRF-positive fibers were increased in the contralateral dorsal horn relative to the naїve and sham control animals. 14 days after SNI, the CRF-positive fibers were also increased in the lamina I of the ipsilateral dorsal horn relative to that observed 7 days after SNI (Table 1, Fig. 3).
In the spinal cords of the naїve and sham control rats, no clearly CRFR1-positive cells were s observed in the spinal cord and DRG. Seven and 14 days after SNI, CRFR1-positive cells were observed in the deeper laminae of the dorsal horn. In the double-labeling staining with Iba1, a marker for microglia, those CRFR1-positive cells were co-stained with Iba1; the results confirmed that CRFR1-positive cells in the deeper laminae were activated microglia (Fig. 4).
Very weak immunoreactivity for CRFR2 was detected in the spinal cord and DRG of the naїve and sham control animals. The intensity and number of CRFR2-immunoreactive cells were increased in the contralateral DRG of the SNI group. The sizes of the CRFR2-positive cells varied from small-sized to medium- and large-sized cells (Table 2, Fig. 5).
In this study, we evaluated the expression of CRF and its receptors in the spinal cord and DRG; the main results are as follows: 1) CRF was normally expressed in the superficial laminae of the dorsal horn and a few small neurons in DRG; 2) After SNI, CRF-immunoreactive neurons in DRG and primary afferent fibers in the spinal dorsal horn were increased on the contralateral side of the injury; 3) CRFR1- and CRFR2-positive cells were not observed in the normal spinal cord and DRG; 4) After SNI, CRFR1 receptors are expressed in the microglia in the deeper lamina of the dorsal horn and CRFR2 receptors were expressed in the contralateral DRG.
The existence of the CRF in DRG and primary afferent fibers in spinal dorsal horns has been demonstrated in previous studies, but the role played by CRF in the pain transmission pathway to antinociception was not defined, because the CRF was shown to exert antinociceptive effects mainly on higher centers, including the HPA axis. Most studies regarding the action of CRF in the spinal cord have focused on visceral sensations. Recently, it was determined in electrophysiological experiments that CRF can modulate sensory transmission by modulating the activity of the spinal neurons [24]. To the best of our knowledge, there have been no reports regarding altered CRF expression in DRG neurons subsequent to peripheral nerve injury. We determined that CRF expression is upregulated in both DRG neurons and primary afferent fibers in the contralateral dorsal horn after SNI. The upregulation of CRF in DRG neurons after SNI may be a response to stress on the peripheral and spinal levels, and may also perform a relevant function in the modulation of pain transmission at the spinal level. However, CRF-positive cells in the DRG ipsilateral to injury were not observed. In our nerve injury model, all peripheral axons of the L5 nerve were cut. The axotomized DRG neuron on the ipsilateral side, and particularly small-sized cells, are known to be susceptible to degenerative and apoptic changes resulting from starvation of neurotrophic factors, prolonged expression of c-Jun transcription factors, and low levels of the cell death repressors Bcl-2 and Bcl-XL [25, 26]. Local upregulation of CRF may occur in the DRG neurons of the contralateral side, but not in the neurons of the ipsilateral side whose axons have been damaged by SNI.
CRFR1 was expressed in the deeper laminae of the dorsal horn and CRFR2 in contralateral DRG neurons after SNI. CRFR1 and R2 evidence widespread distribution in the CNS and other peripheral organs. CRFR1 is expressed in the brain, pituitary gland, gonads, and skin, and CRFR2 is expressed in the brain and the cardiac and skeletal muscles [2]. In the spinal cord, the expression of CRFRs has been detected in neurons in lamina VII and X [16], in cultured microglia, and in activated microglia in paraventricular nucleus [27], as well as in the brain following ischemic damage [28]; however, no expression in the spinal microglia after SNI has yet been reported.
Microglial activation is a key factor in defense mechanisms exploited in response to neuronal injuries such as infection, inflammation, and ischemia [29], but activated microglia are also known to play an active role in the development and maintenance of the neuropathic pain state [20, 30, 31]. Accordingly, the inhibition or attenuation of microglial activation has been considered recently as a possible new tool for the treatment of neuropathic pain induced by spinal nerve or spinal cord injury [32, 33]. Interestingly, a previous report asserted that CRF may regulate neuroinflammation via the induction of microglial apoptosis under in vitro conditions [19]. Upregulated CRF in the DRG neurons has been suggested to act on microglial CRFR1 to modulate microglial activation and neuropathic pain. CRFR2 expression in the DRG neurons was also unexpected. In future studies, the functional significance of this receptor in the neuropathic pain state should be elucidated in greater detail.
In summary, the results of this study suggest that the upregulation of CRFR1 in the microglia occurs as a direct response to peripheral nerve injury and the increased expression of CRF and CRFR2 in DRG neurons, and also may be involved in the mediation of general stress responses in the neuropathic pain state.
Figures and Tables
![]() | Fig. 1Von Frey test for the assessment of mechanical hyperalgesia in the sham control (sham) and spinal nerve injury (SNI) rats. The SNI rats evidenced reductions in the paw withdrawal threshold relative to the sham control group. One-way ANOVA (n=5, *P<0.005 vs. Con, #P<0.005 vs. sham). |
![]() | Fig. 2Corticotropin-releasing factor (CRF) and transient receptor potential vanilloid 1 receptor (TRPV1) expression in the L5 dorsal root ganglion (DRG) of the naїve and spinal nerve injury (SNI) rats. (A) In the DRG of naїve rats, CRF was expressed in a few small neurons, and the majority of CRF-positive neurons also expressed TRPV1 (arrows). (B) After SNI, virtually all immunoreactivity for CRF and TRPV1 disappeared in the ipsilateral (ipsi) DRG. (C) In the contralateral (contra) DRG of the SNI group rats, the levels of CRF-positive neurons were increased relative to that of naїve rats. (D) The number of CRF-positive neurons in ipsilateral and contralateral DRGs in naїve rats and SNI group rats. One-way ANOVA (n=5, *P<0.05 vs. naїve). Scale bar=20 µm. |
![]() | Fig. 3Corticotropin-releasing factor (CRF) expression in the spinal dorsal horn in the naїve and spinal nerve injury (SNI) group rats. (A) In the spinal cords of naїve rats, CRF-immunoreactive fibers and puncta were noted in the superficial laminae of the dorsal horn. (B, C) CRF immunoreactive fibers and puncta were increased in lamina I, II of the contralateral (contra) dorsal horn after SNI. Ipsi, ipsilateral. Scale bars=500 µm (left column), 200 µm (middle column). |
![]() | Fig. 4Corticotropin-releasing factor receptor type 1 (CRFR1) expression in the Iba1-positive microglia in the spinal dorsal horn. (A) Immunoreactivity for CRFR1 was not noted in the dorsal horn of the naїve animals. (B) Immoreactivity for CRFR1 was noted in the activated microglia in the laminae III-V, but not in the lamina I-II (asterisk), of the ipsilateral (ipsi) dorsal horn at 7 days after spinal nerve injury (SNI). (C) At 14 days after SNI, CRF expression was weaker relative to that observed 7 days after SNI. (D) Confocal microscopy for CRFR1 and Iba1 expression in the ipsilateral dorsal horn at 14 days after SNI. CRFR1-positive cells in the ipsilateral dorsal horn were also stained for Iba1 (arrows) in the deeper laminae. Contra, contralateral. Scale bars=500 µm (A-C), 50 µm (D). |
![]() | Fig. 5Expression of corticotropin-releasing factor receptor type 2 (CRFR2) in the L5 dorsal root ganglion (DRG) of the sham control and spinal nerve injury (SNI) group rats. (A, B) In the sham control rats, weak immunoreactivity for CRFR2 was noted in the majority of DRG neurons. (C, D) The increase in CRFR2 immunoreactivity was observed in the neurons (arrows) of the contralateral (contra) DRG after SNI. Ipsi, ipsilateral. Scale bar=200 µm. |
Table 1
The intensity of CRF immunoreactivity in the contralateral and ipsilateral dorsal horns of the naїve rats and the rats 7 days (SNI 7d) and 14 days after SNI (SNI 14d)
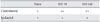
Acknowledgements
This work was supported by a National Research Foundation of Korea (NRF) grant (No: E 00460) funded by the Korean Government.
References
1. Perrin MH, Sutton SW, Cervini LA, Rivier JE, Vale WW. Comparison of an agonist, urocortin, and an antagonist, astressin, as radioligands for characterization of corticotropin-releasing factor receptors. J Pharmacol Exp Ther. 1999. 288:729–734.
2. Perrin MH, Vale WW. Corticotropin releasing factor receptors and their ligand family. Ann N Y Acad Sci. 1999. 885:312–328.
3. Emeric-Sauval E. Corticotropin-releasing factor (CRF): a review. Psychoneuroendocrinology. 1986. 11:277–294.
4. Olschowka JA, O'Donohue TL, Mueller GP, Jacobowitz DM. The distribution of corticotropin releasing factor-like immunoreactive neurons in rat brain. Peptides. 1982. 3:995–1015.
5. Merchenthaler I, Hynes MA, Vigh S, Shally AV, Petrusz P. Immunocytochemical localization of corticotropin releasing factor (CRF) in the rat spinal cord. Brain Res. 1983. 275:373–377.
6. Skofitsch G, Zamir N, Helke CJ, Savitt JM, Jacobowitz DM. Corticotropin releasing factor-like immunoreactivity in sensory ganglia and capsaicin sensitive neurons of the rat central nervous system: colocalization with other neuropeptides. Peptides. 1985. 6:307–318.
7. Kawatani M, Suzuki T, de Groat WC. Corticotropin releasing factor-like immunoreactivity in afferent projections to the sacral spinal cord of the cat. J Auton Nerv Syst. 1996. 61:218–226.
8. De Souza EB. Corticotropin-releasing factor receptors in the rat central nervous system: characterization and regional distribution. J Neurosci. 1987. 7:88–100.
9. Bell JA, de Souza EB. Functional corticotropin-releasing factor receptors in neonatal rat spinal cord. Peptides. 1988. 9:1317–1322.
10. Lariviere WR, Melzack R. The role of corticotropin-releasing factor in pain and analgesia. Pain. 2000. 84:1–12.
11. Amit Z, Galina ZH. Stress-induced analgesia: adaptive pain suppression. Physiol Rev. 1986. 66:1091–1120.
12. Mousa SA, Bopaiah CP, Richter JF, Yamdeu RS, Schäfer M. Inhibition of inflammatory pain by CRF at peripheral, spinal and supraspinal sites: involvement of areas coexpressing CRF receptors and opioid peptides. Neuropsychopharmacology. 2007. 32:2530–2542.
13. Ji G, Neugebauer V. Differential effects of CRF1 and CRF2 receptor antagonists on pain-related sensitization of neurons in the central nucleus of the amygdala. J Neurophysiol. 2007. 97:3893–3904.
14. Fu Y, Neugebauer V. Differential mechanisms of CRF1 and CRF2 receptor functions in the amygdala in pain-related synaptic facilitation and behavior. J Neurosci. 2008. 28:3861–3876.
15. Studeny S, Vizzard MA. Corticotropin-releasing factor (CRF) expression in postnatal and adult rat sacral parasympathetic nucleus (SPN). Cell Tissue Res. 2005. 322:339–352.
16. Korosi A, Kozicz T, Richter J, Veening JG, Olivier B, Roubos EW. Corticotropin-releasing factor, urocortin 1, and their receptors in the mouse spinal cord. J Comp Neurol. 2007. 502:973–989.
17. Sengupta JN. Visceral pain: the neurophysiological mechanism. Handb Exp Pharmacol. 2009. (194):31–74.
18. Cabot PJ. Immune-derived opioids and peripheral antinociception. Clin Exp Pharmacol Physiol. 2001. 28:230–232.
19. Ock J, Lee H, Kim S, Lee WH, Choi DK, Park EJ, Kim SH, Kim IK, Suk K. Induction of microglial apoptosis by corticotropin-releasing hormone. J Neurochem. 2006. 98:962–972.
20. Narita M, Yoshida T, Nakajima M, Narita M, Miyatake M, Takagi T, Yajima Y, Suzuki T. Direct evidence for spinal cord microglia in the development of a neuropathic pain-like state in mice. J Neurochem. 2006. 97:1337–1348.
21. Kim SH, Chung JM. An experimental model for peripheral neuropathy produced by segmental spinal nerve ligation in the rat. Pain. 1992. 50:355–363.
22. Lee SE, Kim JH. Involvement of substance P and calcitonin gene-related peptide in development and maintenance of neuropathic pain from spinal nerve injury model of rat. Neurosci Res. 2007. 58:245–249.
23. Chaplan SR, Bach FW, Pogrel JW, Chung JM, Yaksh TL. Quantitative assessment of tactile allodynia in the rat paw. J Neurosci Methods. 1994. 53:55–63.
24. Ikeda H, Kusudo K, Ryu PD, Murase K. Effects of corticotropin-releasing factor on plasticity of optically recorded neuronal activity in the substantia gelatinosa of rat spinal cord slices. Pain. 2003. 106:197–207.
25. Gillardon F, Klimaschewski L, Wickert H, Krajewski S, Reed JC, Zimmermann M. Expression pattern of candidate cell death effector proteins Bax, Bcl-2, Bcl-X, and c-Jun in sensory and motor neurons following sciatic nerve transection in the rat. Brain Res. 1996. 739:244–250.
26. Zimmermann M. Pathobiology of neuropathic pain. Eur J Pharmacol. 2001. 429:23–37.
27. Du F, Yin L, Shi M, Cheng H, Xu X, Liu Z, Zhang G, Wu Z, Feng G, Zhao G. Involvement of microglial cells in infrasonic noise-induced stress via upregulated expression of corticotrophin releasing hormone type 1 receptor. Neuroscience. 2010. 167:909–919.
28. Stevens SL, Shaw TE, Dykhuizen E, Lessov NS, Hill JK, Wurst W, Stenzel-Poore MP. Reduced cerebral injury in CRH-R1 deficient mice after focal ischemia: a potential link to microglia and atrocytes that express CRH-R1. J Cereb Blood Flow Metab. 2003. 23:1151–1159.
29. Kreutzberg GW. Microglia: a sensor for pathological events in the CNS. Trends Neurosci. 1996. 19:312–318.
30. Hains BC, Waxman SG. Activated microglia contribute to the maintenance of chronic pain after spinal cord injury. J Neurosci. 2006. 26:4308–4317.
31. Schreiber KL, Beitz AJ, Wilcox GL. Activation of spinal microglia in a murine model of peripheral inflammation-induced, long-lasting contralateral allodynia. Neurosci Lett. 2008. 440:63–67.
32. Ledeboer A, Sloane EM, Milligan ED, Frank MG, Mahony JH, Maier SF, Watkins LR. Minocycline attenuates mechanical allodynia and proinflammatory cytokine expression in rat models of pain facilitation. Pain. 2005. 115:71–83.
33. Festoff BW, Ameenuddin S, Arnold PM, Wong A, Santacruz KS, Citron BA. Minocycline neuroprotects, reduces microgliosis, and inhibits caspase protease expression early after spinal cord injury. J Neurochem. 2006. 97:1314–1326.