Abstract
We analyzed aquaporin (AQP) expression in the rat spinal cord following an electrical shock (ES) to elucidate the roles of AQP in spinal cord injury (SCI) induced by an electrical burn. In control animals, AQP1 immunoreactivity was observed in the small diameter dorsal horn fibers of laminae I and II and in astrocytes and neurons in the spinal cord. Both AQP4 and AQP9 immunoreactivity were detected in astrocytes. One week after the ES, AQP1 immunoreactivity in dorsal horn fibers was downregulated to 83, 61, and 33% of control levels following a 1-, 4-, or 6-second ES, respectively. However, AQP1 immunoreactivity in ventral horn neurons increased to 1.3-, 1.5-, and 2.4-fold of control levels following a 1-, 4-, or 6-second ES, respectively. AQP4 immunoreactivity was upregulated after an ES in laminae I and II astrocytes in a stimulus-intensity independent manner. Unlike AQP1 and AQP4, AQP9 immunoreactivity was unaffected by the ES. These findings indicate that altered AQP immunoreactivity may be involved in SCI following an ES.
In cases of electrical burn, electric current flows along the structures with the lowest resistance (e.g., muscle, nerve, and blood vessels) and eventually causes severe heat injury in structures with high resistance (e.g., skin, ligament, and bony tissue) [1, 2]. Some patients show a spinal cord injury following electrical burns. Several clinical studies, including ours, have attempted to elucidate the correlation between pathophysiology and clinical outcomes in patients with a spinal cord injury (SCI) [3, 4]. Interestingly, we have reported that most patients had initial hypotonia between days 2 and 10 after an electrical burn, although an imaging study did not reveal any mechanical injury to the spinal cord [5]. However, few data explain the molecular mechanism of SCI following an electrical burn.
Aquaporins (AQPs) are water channels that provide a major route for water movement across plasma membranes in a variety of tissues including the brain [6-10]. Six AQP subtypes have been described in the normal rat central nervous system (CNS): AQP1, AQP3, AQP4, AQP5, AQP8, and AQP9 [11-15]. Among them, AQP1, AQP4, and AQP9 protein expression levels and localization have been established in the CNS. AQP1 protein expression is restricted to the ventricular-facing surface of the choroid plexus. AQP4 is expressed in astrocyte foot processes near blood vessels and in ependymal and pial surfaces in contact with cerebrospinal fluid. The AQP9 protein has been detected in cells lining the cerebral ventricles, including ependymal cells, tanycytes, and astrocytes [9]. AQP1 is expressed in ependymal cells of the spinal cord lining the central canal, in the sensory fibers of the superficial laminae of the dorsal horn, and in neurons [16, 17]. AQP4 is expressed in astrocytes, although localization of AQP9 in the spinal cord is unclear. Based on these studies, AQPs may be involved in SCI following an electrical burn. Indeed, water accumulation has been documented in the acute phase after a spinal cord contusion [18, 19]. Therefore, we provide the first comprehensive description of the acute changes in AQP expression in the rat spinal cord following an electrical shock (ES).
We utilized the progeny of male Sprague-Dawley rats (7 weeks old) obtained from the Experimental Animal Center, Hallym University, Chuncheon, Korea. The animals were provided with a commercial diet and water ad libitum under controlled temperature, humidity, and lighting conditions (22±2℃, 55±5%, and a 12 : 12 light/dark cycle, respectively). Procedures involving animals and their care were conducted in accordance with our institutional guidelines, which comply with the NIH Guide for the Care and Use of Laboratory Animals (NIH publication no. 80-23, 1996). Additionally, all possible efforts were taken to avoid suffering of the animals and to minimize the number of animals used during the experiment.
An ES was induced with an electroconvulsive shock therapy (ECT) unit (Ugo, Basile, Italy). The ECT unit was a current generator that connected to the rat with non-traumatic clips, and was set to deliver a 1-, 4-, or 6-second (n=5) pulse with a frequency of 299 Hz, a pulse width of 0.9 millisecond, and a current of 99 mA. Rats were given an ES from the right ear to the right hind leg. Control animals were not given an ES. Animals immediately recovered following the ES. Rats were observed 1 hour per day in the open field (top of a laboratory cart with a rim and covered with clean absorbent paper) for general behavior, 1-6 days after the ES. All animals moved slowly with gait abnormalities 6 days after the ES. Control animals did not show any behavioral abnormalities. The animals were perfused transcardially with phosphate-buffered saline (PBS) 1 week after the ES followed by 4% paraformaldehyde in 0.1 M PBS (pH 7.4) under urethane anesthesia (1.5 g/kg, i.p.). The thoracic and lumbar portions of the spinal cord were removed, postfixed in the same fixative for 4 hours, and rinsed in PBS containing 30% sucrose at 4℃ for 2 days. Thereafter, the tissues were frozen and sectioned with a cryostat at 30 µm, and consecutive sections were collected in six-well plates containing PBS.
Free-floating sections were incubated with 3% bovine serum albumin (Sigma-Aldrich, St. Louis, MO, USA) in PBS for 30 minutes at room temperature. The sections were then incubated in primary antibodies (listed below) in PBS containing 0.3% Triton X-100 (Sigma-Aldrich) overnight at room temperature: rabbit anti-AQP1 (1 : 200, Abcam, Cambridge, UK), AQP4 (1 : 200, Chemicon, Temecula, CA, USA) or AQP9 IgG (1 : 200, LifeSpan, Seattle, WA, USA). The sections were washed three times for 10 minutes with PBS, incubated sequentially in biotinylated horse anti-mouse or goat anti-rabbit IgG (Vector, Burlingame, CA, USA) and ABC complex (Vector), diluted 1 : 200 in the same solution as the primary antiserum. The tissues were washed with PBS three times for 10 minutes each between incubations. The sections were visualized with 3,3'-diaminobenzidine (Sigma-Aldrich) in 0.1 M Tris buffer and mounted on gelatin-coated slides. The immunoreactions were observed under an Axiophot microscope (Carl Zeiss, Munchen-Hallbergmoos, Germany), and all images were captured using an Axiocam HRc camera and Axio Vision 3.1 software (Carl Zeiss). Double immunofluorescent staining for AQP1/NeuN or AQP1, AQP4, and AQP9/glial fibrillary acidic protein (GFAP) was also performed. Brain tissues were incubated in a mixture of rabbit anti-AQP1, AQP4, or AQP9 IgG (1 : 50)/mouse anti-GFAP IgG (1 : 100), rabbti anti-AQP1 IgG/mouse anti-NeuN IgG (1 : 200) overnight at room temperature. After washing three times for 10 minutes with PBS, the sections were incubated in a mixture of FITC- and Cy3-conjugated secondary antisera (1 : 200, Amersham Pharmacia Biotech, Piscataway, NJ, USA) for 1 hour at room temperature. Sections were mounted in Vectashield mounting medium (Vector). A negative control test was conducted with preimmune serum instead of the primary antibody (for GFAP), or a pre-absorption test was performed with control peptide (for AQPs) to establish immunostaining specificity. The immunohistochemistry control resulted in the absence of immunoreactivity in any structure (data not shown). All experimental groups were included in each immunochemistry procedure, so they were processed under the same conditions.
Images of each section on the monitor were captured (five sections per patient) to quantify the data. The mean gray value and its standard deviation were obtained from the selected images using Adobe PhotoShop ver. 8.0 (Adobe, San Jose, CA, USA). Each image was normalized by assessing the mean grey value. Intensity measurements were represented as the mean number on a 256 gray scale using NIH Image ver. 1.59 software (National Institute of Health, Bethesda, MD, USA). Background staining values were obtained from neurons. Optical density values were then corrected by subtracting the average background noise values obtained from 15 image inputs. All data obtained from the quantitative measurements were analyzed using a one-way analysis of variance to determine statistical significance. Bonferroni's test was used for post-hoc comparisons. A P<0.05 was considered statistically significant.
In control rats, AQP1 immunoreactivity was observed in the small diameter dorsal horn fibers of laminae I and II. Ependymal cells lining the central canal also showed AQP1 immunoreactivity (data not shown). AQP1 immunoreactivity was also detected in astrocytes within the white and gray matter. However, astrocytes in the white matter showed higher levels of AQP1 immunoreactivity than those in the gray matter (Figs. 1A, 2A, B). AQP1 immunoreactivity was weakly observed in the cell bodies of sensory and motor neurons in the spinal cord. The same result was obtained irrespective of the region of the spinal cord examined (thoracic and lumbar portions). After the ES, AQP1 immunoreactivity in the dorsal horn fibers was downregulated in a stimulus-intensity independent manner. One-, 4-, and 6-second ESs reduced AQP1 immunoreactivity to 83, 61, and 33% of control levels (Fig. 1B-E). Following the ES, AQP1 immunoreactivity was clearly observed in neurons within the ventral horn, although it was rarely detected in neurons of control animals (Fig. 2C). This AQP1 upregulation in neurons was also revealed in a stimulus-intensity dependent manner. After 1-, 4-, and 6-second ESs, the intensity of AQP1 immunoreactivity increased 1.3-, 1.5-, and 2.4-fold in the ventral horn neurons to that observed in controls, respectively (Fig. 1B-E). AQP1 immunoreactivity in astrocytes was unaffected by the ES.
AQP4 was primarily expressed in gray and white matter astrocytes in control animals (Fig. 3A). The same result was obtained irrespective of the region of the spinal cord examined (thoracic and lumbar portions). After the ES, AQP4 immunoreactivity was upregulated in astrocytes within laminae I and II in a stimulus-intensity independent manner. Thus, following the ES, all animals showed increased AQP4 immunoreactivity in astrocytes of laminae I and II to about 1.7-fold of control levels (Figs. 2D, 3B-E). Astrocytes in laminae I and II were not swollen, although they showed strong AQP4 immunoreactivity. AQP4 immunoreactivity in white matter astrocytes and other laminae of the gray matter was unaffected by the ES.
AQP9 was primarily expressed in gray and white matter astrocytes in control animals (Fig. 4A). A double immunofluorescent study revealed that AQP9 immunoreactivity was observed in GFAP astrocytes surrounding some neurons and vessels (Fig. 2E). The same result was obtained irrespective of the region of the spinal cord examined (thoracic and lumbar portions). Unlike AQP1 and AQP4, AQP9 immunoreactivity was unaffected by the ES (Fig. 4B-E).
AQPs have been subdivided into three groups based on their permeability characteristics [20]: AQP0, AQP1, AQP2, AQP4, AQP5, and AQP6 are permeable to water; AQP3, AQP7, and AQP8 are permeable to water, glycerol, and urea (aquaglyceroporins); and AQP9 is permeable to water, glycerol, urea, purines, pyrimidines, and monocarboxylates (neutral solute channel). Thus, AQPs are not only implicated in water movement during edema formation, but also play a role as a metabolite channel in the CNS [21, 22]. In the brain, AQP1 is upregulated in astrocytes following various disruptions, including subarachnoid hemorrhage, transient focal ischemia, Alzheimer's disease, traumatic brain injury, and the presence of hemangioblastomas, although their expression is rarely observed in normal brain [11, 23-27]. In contrast to the brain, AQP1 is apparently expressed in the small diameter dorsal horn fibers and astrocytes [28]. Considering the expression of AQP1 in the dorsal horn, it has been suggested that AQP1 plays a role in normal pain processing, although the effect of AQP1 knockout on pain regulation is controversial [17, 29]. Furthermore, Oshio et al. [30] have reported that a large population of dorsal root ganglion neurons express AQP1 mRNA and protein, and that these neurons are co-labeled for markers of presumed nociceptors, including substance P. Strikingly, the present study showed downregulated AQP1 expression in dorsal horn fibers but upregulated AQP1 immunoreactivity in the ventral horn neurons. Nesic et al. [28] reported that a rat contusion SCI induces persistent and significant increases in AQP1 at lesions and proposed that AQP1 overexpression may play a role in the development of neuropathic pain after SCI. Based on this previous report, our findings indicate that downregulation of AQP1 immunoreactivity in the dorsal horn may be a compensatory response to reduce ES-induced pain. In contrast to the dorsal horn, overexpression of AQP1 immunoreactivity in neurons within the ventral horn may be an apoptotic event, because Jablonski et al. [31] reported that AQP1-mediated water loss is important for apoptotic volume decreases and downstream apoptotic events, and that plasma membrane water permeability can control the rate of apoptosis.
AQP4 is a principal water permeability molecule in the brain [32, 33]. Following brain injury or SCI, alterations in AQP4 expression are associated with an accumulation of water, which induces edema [34]. In the present study, AQP4 immunoreactivity was upregulated in astrocytes of laminae I and II in a stimulus-intensity independent manner following an ES. These findings are simply a speculation that AQP4 expression in laminae I and II could be an initiator of cytotoxic edema formation induced by an ES. Because astroglial swelling is the main mediator of brain edema [35], increased AQP4 expression would worsen cytotoxic edema. Indeed, AQP-null mice show improved cytotoxic edema [36]. In the present study, astrocytes in laminae I and II were not swollen, although they showed strong AQP4 immunoreactivity. Furthermore, AQP4 immunoreactivity in white matter astrocytes and other gray matter laminae was unaffected by the ES. Therefore, upregulated AQP4 expression in astrocytes within laminae I and II would not be related to ES-induced cytotoxic edema formation. In contrast, AQP4 knockout mice show involvement of AQP4 during astrocyte migration [33, 37]. An AQP4 deficiency delays astrocyte migration in response to a chemotactic stimulus in vitro, and AQP4 deletion inhibits glial scar progression following injury in vivo [37]. Indeed, nerve fibers in the superficial laminae of the dorsal horns are capable of sprouting and undergoing structural reorganization that results in neuropathic pain [28, 38]. During this processing, astroglia play a role guiding nerve fiber sprouting [39]. Based on these studies, upregulated AQP4 immunoreactivity may be related to astroglial cell migration in laminae I and II, which participate in axonal reorganization following an ES. Alternatively, upregulated AQP4 immunoreactivity in astrocytes may be morphological evidence of ES-induced pain, because the AQP4 mRNA level increases significantly in central neuropathic pain rats [40]. Taken together, AQP4 upregulation in astrocytes may be involved in ES-induced pain.
AQP9 immunoreactivity is rarely expressed in the normal brain, but its immunoreactivity is upregulated following various CNS insults [11, 26, 27]. Metabolic disturbances during insults induce accumulations of CO2 and lactic acid from active cells, resulting in extracellular acidification [41-44]. In this condition, AQP9 in astrocytes is involved in the elimination of excess glycerol and lactate from the extracellular space [27]. AQP9 permeability to lactate increases 4-fold when pH decreases to 5.5 [45]. Therefore, lactic acidosis increases AQP9 permeability and enables the uptake of excess lactate by astrocytes [27]. Indeed, our previous study revealed upregulation of AQP9 expression in hippocampal astrocytes following pilocarpine-induced status epilepticus. Unexpectedly, the results of the present study showed that AQP9 was primarily expressed in gray and white matter astrocytes in control animals but was unaffected by the ES. These findings indicate that, unlike brain, AQP9 may distribute and play a role in functioning of the normal spinal cord, although the exact biological role is still unknown. Furthermore, AQP9 may not be involved in neuropathic pain following an ES.
Previously, we reported that most electrical burn patients were initially noted to have hypotonia between days 2 and 10 after the electrical burn and were characterized by ascending paralysis, i.e., paraplegia followed by quadriplegia. We have postulated that these unique neurological manifestations after an electrical injury may be related to an insufficient blood supply to the spinal cord [5]. Furthermore, it is noteworthy that changes in AQP expression are correlated with cytotoxic and vasogenic edema following chemically induced seizure activity [46, 47]. Taken together, our results suggest that acute changes in AQP expression in the spinal cord may be involved in neurological manifestations in patients suffering from electrical burns. However, further study is needed to fully develop this hypothesis.
In conclusion, AQP subunits may play different roles in ES-induced pain. Therefore, our findings suggest that selective regulation of AQP subunit functions may provide new therapeutic approaches to electrical injury of the spinal cord.
Figures and Tables
Fig. 1
Aquaporin (AQP)1 immunoreactivity in rat spinal cord following an electrical shock (ES). (A) Control. (B) 1 s ES. (C) 4 s ES. (D) 6 s ES. In control rats, AQP1 immunoreactivity was observed in the small diameter dorsal horn fibers in laminae I and II. After the ES, AQP1 immunoreactivity in dorsal horn fibers was downregulated in a stimulus-intensity dependent manner (arrows, column 2). However, AQP1 immunoreactivity in neurons within the ventral horn was clearly upregulated in a stimulus-intensity dependent manner (arrows, column 3). Rectangles in column 1 indicate a region in columns 2 and 3, respectively. Scale bar=400 µm (column 1), 50 µm (columns 2, 3). (E) Quantitative analyses of AQP1 in the spinal cord following an ES (mean±SEM). Significant differences from control animals, *P<0.05, **P<0.01.
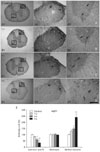
Fig. 2
Double immunofluorescent staining for aquaporins (AQPs), NeuN, or glial fibrillary acidic protein (GFAP) in the spinal cord. AQP1 immunoreactivity (A1, arrow) was not detected in NeuN-positive dorsal horn neurons of the 6-s electrical shock (ES) animals (A2, arrow). In the ventral horn, AQP1 immunoreactivity (B1, C1) was observed in GFAP astrocytes (B2, arrows) and NeuN-positive neurons (C2, arrows). AQP4 (D1, arrows) and AQP9 (E1, arrows) immunoreactivity was detected in GFAP astrocytes (D2, E2, arrows). Blue (column 3) is DAPI counterstaining. Column 4 is a merged-image. Scale bar=12.5 µm.
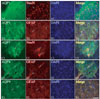
Fig. 3
Aquaporin (AQP)4 immunoreactivity in the rat spinal cord following an electrical shock (ES). (A) Control. (B) 1 s ES. (C) 4 s ES. (D) 6 s ES. After the ES, AQP4 immunoreactivity was upregulated in astrocytes within laminae I and II in a stimulus-intensity independent manner (column 2). However, AQP4 immunoreactivity in the ventral horn was unaffected by the ES. Rectangles in column 1 indicate a region in columns 2 and 3, respectively. Scale bar=400 µm (column 1), 50 µm (columns 2, 3). (E) Quantitative analyses of AQP4 in the spinal cord following an ES (mean±SEM). Significant differences from control animals, *P<0.05, **P<0.01.
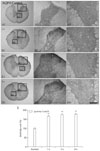
Fig. 4
Aquaporin (AQP)9 immunoreactivity in the rat spinal cord following an electrical shock (ES). (A) Control. (B) 1 s ES. (C) 4 s ES. (D) 6 s ES. Unlike AQP1 and AQP4, AQP9 immunoreactivity was unaffected by the ES. Rectangles in column 1 indicate a region in columns 2 and 3, respectively. Scale bar=400 µm (column 1), 50 µm (columns 2, 3). (E) Quantitative analyses of AQP9 in the spinal cord following an ES (mean±SE). Significant differences from control animals.
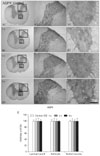
Acknowledgements
This study was supported by a grant of the Korea Healthcare Technology R&D Project, Ministry for Health, Welfare and Family Affairs, Republic of Korea (Grant number A084589).
References
1. Skoog T. Electrical injuries. J Trauma. 1970. 10:816–830.
2. Baxter CR. Present concepts in the management of major electrical injury. Surg Clin North Am. 1970. 50:1401–1418.
3. Xiang D, Shen Z. Experimental high-voltage electrical burns on limbs of rabbits. Zhonghua Zheng Xing Shao Shang Wai Ke Za Zhi. 1998. 14:429–432.
4. Segal JL, Maltby BF, Langdorf MI, Jacobson R, Brunnemann SR, Jusko WJ. Methylprednisolone disposition kinetics in patients with acute spinal cord injury. Pharmacotherapy. 1998. 18:16–22.
5. Ko SH, Chun W, Kim HC. Delayed spinal cord injury following electrical burns: a 7-year experience. Burns. 2004. 30:691–695.
6. Agre P, King LS, Yasui M, Guggino WB, Ottersen OP, Fujiyoshi Y, Engel A, Nielsen S. Aquaporin water channels: from atomic structure to clinical medicine. J Physiol. 2002. 542(Pt 1):3–16.
7. Verkman AS. Role of aquaporins in endothelial water transport. J Anat. 2002. 200:528.
8. Manley GT, Fujimura M, Ma T, Noshita N, Filiz F, Bollen AW, Chan P, Verkman AS. Aquaporin-4 deletion in mice reduces brain edema after acute water intoxication and ischemic stroke. Nat Med. 2000. 6:159–163.
9. Badaut J, Lasbennes F, Magistretti PJ, Regli L. Aquaporins in brain: distribution, physiology, and pathophysiology. J Cereb Blood Flow Metab. 2002. 22:367–378.
10. Amiry-Moghaddam M, Ottersen OP. The molecular basis of water transport in the brain. Nat Rev Neurosci. 2003. 4:991–1001.
11. Badaut J, Hirt L, Granziera C, Bogousslavsky J, Magistretti PJ, Regli L. Astrocyte-specific expression of aquaporin-9 in mouse brain is increased after transient focal cerebral ischemia. J Cereb Blood Flow Metab. 2001. 21:477–482.
12. Elkjaer M, Vajda Z, Nejsum LN, Kwon T, Jensen UB, Amiry-Moghaddam M, Frøkiaer J, Nielsen S. Immunolocalization of AQP9 in liver, epididymis, testis, spleen, and brain. Biochem Biophys Res Commun. 2000. 276:1118–1128.
13. Nielsen S, Nagelhus EA, Amiry-Moghaddam M, Bourque C, Agre P, Ottersen OP. Specialized membrane domains for water transport in glial cells: high-resolution immunogold cytochemistry of aquaporin-4 in rat brain. J Neurosci. 1997. 17:171–180.
14. Venero JL, Vizuete ML, Ilundáin AA, Machado A, Echevarria M, Cano J. Detailed localization of aquaporin-4 messenger RNA in the CNS: preferential expression in periventricular organs. Neuroscience. 1999. 94:239–250.
15. Yamamoto N, Yoneda K, Asai K, Sobue K, Tada T, Fujita Y, Katsuya H, Fujita M, Aihara N, Mase M, Yamada K, Miura Y, Kato T. Alterations in the expression of the AQP family in cultured rat astrocytes during hypoxia and reoxygenation. Brain Res Mol Brain Res. 2001. 90:26–38.
16. Oshio K, Watanabe H, Song Y, Verkman AS, Manley GT. Reduced cerebrospinal fluid production and intracranial pressure in mice lacking choroid plexus water channel Aquaporin-1. FASEB J. 2005. 19:76–78.
17. Shields SD, Mazario J, Skinner K, Basbaum AI. Anatomical and functional analysis of aquaporin 1, a water channel in primary afferent neurons. Pain. 2007. 131:8–20.
18. Li S, Tator CH. Effects of MK801 on evoked potentials, spinal cord blood flow and cord edema in acute spinal cord injury in rats. Spinal Cord. 1999. 37:820–832.
19. Sharma HS, Badgaiyan RD, Alm P, Mohanty S, Wiklund L. Neuroprotective effects of nitric oxide synthase inhibitors in spinal cord injury-induced pathophysiology and motor functions: an experimental study in the rat. Ann N Y Acad Sci. 2005. 1053:422–434.
20. Verkman AS, Mitra AK. Structure and function of aquaporin water channels. Am J Physiol Renal Physiol. 2000. 278:F13–F28.
21. Badaut J, Petit JM, Brunet JF, Magistretti PJ, Charriaut-Marlangue C, Regli L. Distribution of Aquaporin 9 in the adult rat brain: preferential expression in catecholaminergic neurons and in glial cells. Neuroscience. 2004. 128:27–38.
22. Badaut J, Regli L. Distribution and possible roles of aquaporin 9 in the brain. Neuroscience. 2004. 129:971–981.
23. Pérez E, Barrachina M, Rodríguez A, Torrejón-Escribano B, Boada M, Hernández I, Sánchez M, Ferrer I. Aquaporin expression in the cerebral cortex is increased at early stages of Alzheimer disease. Brain Res. 2007. 1128:164–174.
24. Rodríguez A, Pérez-Gracia E, Espinosa JC, Pumarola M, Torres JM, Ferrer I. Increased expression of water channel aquaporin 1 and aquaporin 4 in Creutzfeldt-Jakob disease and in bovine spongiform encephalopathy-infected bovine-PrP transgenic mice. Acta Neuropathol. 2006. 112:573–585.
25. Chen Y, Tachibana O, Oda M, Xu R, Hamada J, Yamashita J, Hashimoto N, Takahashi JA. Increased expression of aquaporin 1 in human hemangioblastomas and its correlation with cyst formation. J Neurooncol. 2006. 80:219–225.
26. Badaut J, Brunet JF, Grollimund L, Hamou MF, Magistretti PJ, Villemure JG, Regli L. Aquaporin 1 and aquaporin 4 expression in human brain after subarachnoid hemorrhage and in peritumoral tissue. Acta Neurochir Suppl. 2003. 86:495–498.
27. Ribeiro M, Beleza P, Fernandes J, Almeida F, Rocha J. Reverse crossed cerebellar diaschisis. Acta Med Port. 2006. 19:439–441.
28. Nesic O, Lee J, Unabia GC, Johnson K, Ye Z, Vergara L, Hulsebosch CE, Perez-Polo JR. Aquaporin 1: a novel player in spinal cord injury. J Neurochem. 2008. 105:628–640.
29. Oshio K, Song Y, Verkman AS, Manley GT. Aquaporin-1 deletion reduces osmotic water permeability and cerebrospinal fluid production. Acta Neurochir Suppl. 2003. 86:525–528.
30. Oshio K, Watanabe H, Yan D, Verkman AS, Manley GT. Impaired pain sensation in mice lacking Aquaporin-1 water channels. Biochem Biophys Res Commun. 2006. 341:1022–1028.
31. Jablonski E, Webb A, Hughes FM Jr. Water movement during apoptosis: a role for aquaporins in the apoptotic volume decrease (AVD). Adv Exp Med Biol. 2004. 559:179–188.
32. Ma T, Yang B, Gillespie A, Carlson EJ, Epstein CJ, Verkman AS. Generation and phenotype of a transgenic knockout mouse lacking the mercurial-insensitive water channel aquaporin-4. J Clin Invest. 1997. 100:957–962.
33. Verkman AS, Binder DK, Bloch O, Auguste K, Papadopoulos MC. Three distinct roles of aquaporin-4 in brain function revealed by knockout mice. Biochim Biophys Acta. 2006. 1758:1085–1093.
34. Unterberg AW, Stover J, Kress B, Kiening KL. Edema and brain trauma. Neuroscience. 2004. 129:1021–1029.
35. Kimelberg HK. Current concepts of brain edema: review of laboratory investigations. J Neurosurg. 1995. 83:1051–1059.
36. Manley GT, Binder DK, Papadopoulos MC, Verkman AS. New insights into water transport and edema in the central nervous system from phenotype analysis of aquaporin-4 null mice. Neuroscience. 2004. 129:983–991.
37. Saadoun S, Papadopoulos MC, Watanabe H, Yan D, Manley GT, Verkman AS. Involvement of aquaporin-4 in astroglial cell migration and glial scar formation. J Cell Sci. 2005. 118(Pt 24):5691–5698.
38. Nacimiento W, Töpper R, Fischer A, Oestreicher AB, Nacimiento AC, Gispen WH, Noth J, Kreutzberg GW. Immunocytochemistry of B-50 (GAP-43) in the spinal cord and in dorsal root ganglia of the adult cat. J Neurocytol. 1993. 22:413–424.
39. Perosa SR, Porcionatto MA, Cukiert A, Martins JR, Passeroti CC, Amado D, Matas SL, Nader HB, Cavalheiro EA, Leite JP, Naffah-Mazzacoratti MG. Glycosaminoglycan levels and proteoglycan expression are altered in the hippocampus of patients with mesial temporal lobe epilepsy. Brain Res Bull. 2002. 58:509–516.
40. Nesic O, Lee J, Johnson KM, Ye Z, Xu GY, Unabia GC, Wood TG, McAdoo DJ, Westlund KN, Hulsebosch CE, Regino Perez-Polo J. Transcriptional profiling of spinal cord injury-induced central neuropathic pain. J Neurochem. 2005. 95:998–1014.
41. Chesler M, Kaila K. Modulation of pH by neuronal activity. Trends Neurosci. 1992. 15:396–402.
42. Xiong ZQ, Stringer JL. Extracellular pH responses in CA1 and the dentate gyrus during electrical stimulation, seizure discharges, and spreading depression. J Neurophysiol. 2000. 83:3519–3524.
43. Melø TM, Nehlig A, Sonnewald U. Metabolism is normal in astrocytes in chronically epileptic rats: a (13)C NMR study of neuronal-glial interactions in a model of temporal lobe epilepsy. J Cereb Blood Flow Metab. 2005. 25:1254–1264.
44. Dubé C, da Silva Fernandes MJ, Nehlig A. Age-dependent consequences of seizures and the development of temporal lobe epilepsy in the rat. Dev Neurosci. 2001. 23:219–223.
45. Tsukaguchi H, Weremowicz S, Morton CC, Hediger MA. Functional and molecular characterization of the human neutral solute channel aquaporin-9. Am J Physiol. 1999. 277(5 Pt 2):F685–F696.
46. Kim JE, Ryu HJ, Yeo SI, Seo CH, Lee BC, Choi IG, Kim DS, Kang TC. Differential expressions of aquaporin subtypes in astroglia in the hippocampus of chronic epileptic rats. Neuroscience. 2009. 163:781–789.
47. Kim JE, Yeo SI, Ryu HJ, Kim MJ, Kim DS, Jo SM, Kang TC. Astroglial loss and edema formation in the rat piriform cortex and hippocampus following pilocarpine-induced status epilepticus. J Comp Neurol. 2010. 518:4612–4628.