Abstract
The reaction of neuroactive substances to ischemic conditions in the rat retina evoked by different methods was immunochemically evaluated in adult Sprague-Dawley rats. Ocular ischemic conditions were unilaterally produced by elevating intraocular pressure (EIOP) or by middle cerebral artery occlusion (MCAO). Two EF-hand calcium binding proteins, calbindin D28K (CB) and calretinin (CR), in the normal retina showed similar immunolocalization, such as the amacrine and displaced amacrine cells, the ganglion cells, and their processes, particularly CB in horizontal cells. CB immunoreactive neurons in the ganglion cell layer in both types of ischemic retinas were more reduced in number than CR neurons compared to those in a normal retina. The CB protein level in both ischemic retinas was reduced to 60-80% of normal. The CR protein level in MCAO retinas was reduced to about 80% of normal but increased gradually to the normal value, whereas that in the EIOP showed a gradual reduction and a slight recovery. SMI32 immunoreactivity, which detects a dephosphorylated epitope of neurofilaments-M and -H, appeared in the axon bundles of ganglion cells in the innermost nerve fiber layer of normal retinas. The reactivity in the nerve fiber bundles appeared to only increase slightly in EIOP retinas, whereas a moderate increase occurred in MCAO retinas. The SMI32 protein level in MCAO retinas showed a gradual increasing tendency, whereas that in the EIOP showed a slight fluctuation. Interestingly, the MCAO retinas showed additional SMI32 immunoreactivity in the cell soma of presumed ganglion cells, whereas that of EIOP appeared in the Müller proximal radial fibers. Glial fibrillary acidic protein (GFAP) immunoreactivity appeared in the astrocytes located in the nerve fiber layer of normal retinas. Additional GFAP immunoreactivity appeared in the Müller glial fibers deep in EIOP retinas and at the proximal end in MCAO retinas. These findings suggest that the neurons in the ganglion cell layer undergo degenerative changes in response to ischemia, although EIOP retinas represented a remarkable Müller glial reaction, whereas MCAO retinas had only a small-scaled axonal transport disturbance.
The retina is part of the central nervous system and, as an expansion of the diencephalon, acts as a special sense organ to capture images and process and transmit visual information. The incoming information is collected and transmitted from electrical signals into chemical information by photoreceptor cells. It then passes into the final output neurons, the ganglion cells, via synaptic inputs from bipolar cells and amacrine cells, and subsequently encodes action potentials, which are transmitted to higher visual centers along the optic nerve, consisting of ganglion cell axon bundles. Vascular networks are well organized in the retina for such dynamic and delicate activity.
Glaucoma is recognized as a major ocular neurodegenerative disease leading to blindness. Excess aqueous humor by overproduction or by blockade of drainage channels evokes elevated intraocular pressure (EIOP) and subsequently results in ischemic or mechanical injury to optic nerve axons at the level of the optic nerve head. Pathogenic events of a glaucomatous retina are characterized by optic disc cupping and visual field loss [1-3]. A pressure-induced ischemia injury model is commonly used among numerous animal glaucoma models. Such a transient retinal ischemia model system presents a variety of panoramic ischemia effects such as the glial reaction [4, 5] and neuronal degenerative responses including neuronal death and regulated expression of neuroactive substances [6-11]. It is generally accepted that glutamate is the key excitotoxicity molecule during retinal ischemia, because of results in which glutamate is released and initiates the death of neurons containing the ionotropic glutamate N-methyl-D-aspartate (NMDA) receptor [12-14]. Furthermore, elevated glutamate levels have been recorded in the vitreous humor of patients with glaucoma, and NMDA receptors exist on ganglion cells and a subset of amacrine cells. The major cell death cascades after activation of NMDA receptors are the influx of calcium into cells and free radical generation [15]. Based on these data, β-adrenoreceptor blockers have been developed and tested for clinical use in glaucoma as an efficient therapeutic agent capable of calcium channel blocking activity and reducing intraocular pressure. In contrast, normal tension glaucoma without an EIOP is increasing, particularly in Korean and Japanese populations, whereas academic and clinical efforts for treating glaucoma are largely engaged in hypertension glaucoma. More intensive studies must be conducted on the pathophysiology of this glaucoma, as the only proven treatable risk factor for glaucoma is intraocular pressure [16].
In the present study, we aimed to examine the differential pathophysiology between these two glaucomatous states by evaluating and comparing changes in neuroactive substances using immunochemical techniques. A hypertensive glaucoma model was created using the pressure-induced EIOP method, and a normotensive model was created by ischemia via middle cerebral artery occlusion (MCAO). The major target cells of glaucomatous damage are retinal ganglion cells and their major intracellular pathogenic cascades are excess calcium ion influx and axonal transport disturbances. Therefore, we immunochemically evaluated the alteration in calcium binding proteins (calbindin and calretinin), a dephosphorylated form of neurofilament (NF)-M and NF-H expression, and glial responses through glial fibrillary acidic protein (GFAP) expression.
Adult male Sprague-Dawley rats (8-weeks-old) were used. All experimental procedures conformed to the guidelines of the Catholic University ethics committee and the National Institute of Health Guide for the Care and Use of Laboratory Animals (NIH publication number 80-23, revised 1996).
Animals were deeply anesthetized with 4% chloral hydrate (1 ml/100 g body weight). For the EIOP model, the pupils of the right eyeballs were dilated with 1% tropicamide drops and then a 30-gauge needle connected to a hydrostatic pressure device was introduced into the anterior chamber. IOP was increased to 100-120 mm Hg by tuning the device, and the pressure was maintained for 60 minutes. The cannula was then removed to allow recirculation in the retinal blood vessels. The contra lateral eyeballs were treated as experimental controls.
MCAO was performed by the intraluminal thread method designed by Longa et al. [17]. Briefly, the right external carotid artery was tied with a 4-0 silk suture, and a 3-0 rounded tip nylon suture was inserted into the right common carotid artery. The suture was advanced through the internal carotid artery to occlude the MCA and maintained for 60 minutes. The blood flow was then re-circulated by withdrawing the suture from the common carotid artery. During the experiment, body temperatures were maintained at 37.5±0.3℃ with a heating lamp.
Animals were sacrificed with an overdose of chloral hydrate at 1, 3, 5, 7, and 14 days, and five animals were used for each experimental time point including the normal state. Eyeballs were enucleated and opened with an encircling cut along the posterior margin of the ora serrata. For Western blot analysis, the retinas were quickly dissected out of the posterior segments on an ice-cold plate, frozen in liquid nitrogen, and stored at -70℃. For the immunohistochemical analysis, the retinas from the posterior eyecups were fixed in 4% paraformaldehyde in 0.1 M phosphate buffer (PB), pH 7.4, for 2 hours. After fixation, the retinas were carefully dissected and transferred to 30% sucrose (in PB) for 24 hours at 4℃. They were frozen in liquid nitrogen and stored at -70℃.
Retinas were thawed and trimmed into equatorial plane quadrants. They were rinsed in 0.01 M phosphate buffered saline (PBS, pH 7.4), dehydrated, embedded in wax, and cut into 7-µm vertical sections. For blocking nonspecific binding sites, the retinal tissues were first treated with 10% normal donkey serum (Jackson ImmunoResearch, West Grove, PA, USA) in PB with 0.5% Triton X-100. Primary antibodies were incubated at 4℃ overnight, rinsed with PBS for 45 minutes (3×15 minutes) and secondary antibodies were incubated at 4℃ for 2 hours. The primary antibodies were rabbit polyclonal anti-calbindin D28K (CB, 1 : 500, Swant, Bellinzona, Switzerland), goat polyclonal anti-calretinin (CR, 1 : 2,000, Millipore, Temecula, CA, USA), mouse monoclonal anti-SMI32 (1 : 1,000, Covance, Emeryville, CA, USA), for detecting the NF-M and NF-H dephosphorylated epitopes, and mouse monoclonal anti-GFAP (1 : 500, Millipore). The appropriate secondary antibodies were peroxidase conjugated donkey anti-mouse IgG, donkey anti-rabbit IgG, and donkey anti-goat IgG (1 : 100, Jackson ImmunoResearch). Sections were rinsed in two changes of PBS and three changes of 0.05 M Tris-HCl buffer (TB), pH 7.4, for 5 minutes. The color reaction was visualized by incubating with 0.05% 3,3' diaminobenzidine tetrahydrochloride plus 0.01% hydrogen peroxide in TB for 1 minute.
Quantitative values of CB and CR immunoreactive neuronal populations in the ganglion cell layer (GCL) were measured in 7-µm thick retinal sections of normal, EIOP, and MCAO ischemic animals.
The density of CB and CR immunoreactive neurons was calculated in terms of cell numbers appearing in 7-µm thick, 1-mm long sections using a montage of microphotographs taken at ×200 to give representative mean values. At least ten retinal sections from each animal were chosen to quantitatively evaluate these immunoreactive neuronal densities. Data are presented as means ± standard deviations (SDs).
Retinas at each experimental time point were homogenized in ten volumes of sample buffer (20 mM TB, pH 7.4; 150 mM NaCl; 1 mM ethylenediaminetetraacetic acid; 1% Triton X-100; 0.5% sodium deoxycholate; 0.1% sodium dodecyl sulfate [SDS]; 0.02% sodium azide; 1 mM phenylmethanesulfonylfluoride; and 5 µg/ml leupeptin). Protein concentrations were determined in supernatants using the Lowry method [18, 19]. An equal amount of protein (15 µg) was heated to 100℃ for 10 minutes with an equivalent volume of double-strength sample buffer (containing 4% SDS and 10% mercaptoethanol) and loaded onto 10% (for SMI-32) or 15% (for calcium binding proteins) polyacrylamide gels. The proteins were electrotransferred to a nitrocellulose membrane in Tris-glycine-methanol buffer. The membrane was treated in a blocking solution containing 5% skim milk, 0.01% Tween-20, and PBS at room temperature for 1 hour and then incubated with primary antibodies in the blocking solution at 4℃ for 15 hours. The primary antibodies used in the Western blot were rabbit polyclonal anti-CB (1 : 5,000, Swant), goat polyclonal anti-CR (1 : 10,000, Millipore), mouse monoclonal anti-SMI32 (1 : 2,500, Covance), and mouse monoclonal anti-β-actin (1 : 100,000, Sigma, St. Louis, MO, USA) as a control. The membrane was rinsed three times with 0.05% Tween-20 in PBS for 10 minutes each, followed by an incubation in biotinylated donkey anti-mouse IgG, anti-rabbit IgG, or anti-goat IgG (Jackson ImmunoResearch) at room temperature for 1 hour. The immunoblots were washed three times for 10 minutes each and then processed using an Enhanced Chemiluminescence Detection Kit (GE Healthcare, Buckinghamshire, UK). The protein content was calculated by measuring the peak densitometry area with Multi Gauge ver. 3.0 (Fujifilm, Tokyo, Japan). The optical density (mean±SD) was obtained from five determinations for each band. Statistical evaluation was based on Student's t-tests. A P<0.05 indicated statistical significance.
The retina was light microscopically organized into three nuclear layers and two plexiform layers, such as the outer nuclear layer, the outer plexiform layer, the inner nuclear layer (INL), the inner plexiform layer (IPL), and the GCL, in order from the outside. Morphological observations revealed a reduction in thickness of the entire depth of the retina due to thinning of the GCL, IPL, and INL in EIOP ischemic retinas, whereas no large change was observed in MCAO retinas.
Retinal neuronal responses to the two ischemic conditions were analyzed focusing on the neurons in the GCL by anti-CB, anti-CR, and anti-SMI32 immunohistochemistry, and glial response was analyzed by anti-GFAP immunohistochemistry focusing on the Müller glial cells in the vertical sections taken from the same areas as the experimental retinas to reduce experimental error.
CB immunoreactivity was localized to the horizontal cells and amacrine cells in the INL, the displaced amacrine cells and ganglion cells in the GCL, and nerve fiber bundles in the nerve fiber layer (NFL) of the normal retina (Fig. 1A). CB immunoreactivity in the GCL was clearly reduced in the cell bodies of both EIOP (Fig. 1B, C) and MCAO retinas (Fig. 1D-F). CB immunoreactive neurons in the GCL of EIOP retinas were reduced earlier but increased in cell number at later experimental periods (Fig. 2). In the MCAO retinas, the CB neuronal population in the GCL showed the same fluctuations along the experimental time periods (Fig. 2).
CR immunoreactivity was localized to the amacrine cells in the INL and their processes in three bands in the IPL and to the displaced amacrine cells and ganglion cells in the GCL of the normal retina (Fig. 3A). CR immunoreactivity in the GCL was reduced in the neuronal population of both EIOP (Fig. 3B, C) and MCAO retinas (Fig. 3D-F), whereas that increased in the nerve fiber bundles, particularly in the later periods of MCAO (Fig. 3E, F). The number of CR immunoreactive neurons in the GCL of EIOP retinas was reduced to approximately 70% of the normal value at earlier experimental periods but the cell number increased nearly to the normal value at later periods (Fig. 4). These changing patterns of CR immunoreactive GCL neurons during the experimental time periods were similar in EIOP and MCAO retinas (Fig. 4).
SMI32 immunoreactivity was localized to the axon bundles of the ganglion cells in the NFL and to the end feet of the Müller cells in normal retinas. Furthermore, SMI32 immunoreactivity appeared in the proximal radial processes of Müller cells in EIOP retinas (Fig. 5A, B). Interestingly, SMI32 immunoreactivity in MCAO retinas was stronger in the axon bundles and additionally localized to the cell bodies of some ganglion cells and even their dendritic arbors in the INL (Fig. 5C, D).
Changes in CB, CR, and SMI32 proteins in response to the two ischemic conditions were analyzed quantitatively.
CB protein levels in EIOP retinas decreased by more than 60% of normal values and were maintained with no large fluctuations (Fig. 6A). CB protein levels in MCAO retinas decreased to approximately 80% at 1 day and, thereafter, recorded no large fluctuations compared to levels at 1 day (Fig. 6A).
CR protein levels in EIOP retinas gradually decreased to 50% of normal values at the mid-period and recovered to about 90% of normal (Fig. 6B). CR protein levels in MCAO retinas decreased slightly to about 80% of their normal values at earlier time periods and recovered to near normal levels (Fig. 6B).
SMI32 protein levels in EIOP retinas decreased in a shallow U curve to approximately 70% of normal levels throughout the experiment (Fig. 6C). In contrast, the SMI32 level in MCAO retinas decreased to 50% of normal at 1 day and, thereafter, increased gradually to more than the normal value during the last time period (Fig. 6C).
GFAP is generally used as a astrocyte identification marker protein or for detecting the glial reaction to injury stimuli. GFAP immunoreactivity was localized to astrocytes and/or end feet of Müller cells in the retina during the normal state. However, GFAP immunoreactivity showed a different localization pattern according to the ischemia induction mode in ischemic retinas. In EIOP retinas, GFAP immunoreactivity was localized to the end feet at 1 day and, thereafter, into the entire Müller cell profile (Fig. 7A, B). In contrast, immunoreactivity in MCAO ischemic retinas was localized to astrocytes and/or end feet of Müller cells and limited to the innermost end of the retina (Fig. 7C, D).
The present study demonstrated that retinal neuronal degeneration caused by ischemia can occur differentially via different triggering pathways, according to the hypertensive condition. That is, neuronal degeneration in pressure-induced EIOP retinas may be exacerbated due to the accompanying glial reaction, whereas in simple bloodflow blockade-induced MCAO, ischemic retinas occurred due to an axonal transport disturbance.
In the present study, CB and CR, belonging to a family of EF-hand motif calcium binding proteins that were immunolocalized to amacrine cells, displaced amacrine cells, ganglion cells, and horizontal cells in the case of CB, in agreement with other studies [8-10, 20-22]. The expression of these two calcium binding proteins was downregulated in both ischemic retinas. Neuronal responses were evaluated focusing on the GCL neurons, because ischemic stimuli inducing both arrive at the nerve fiber layer, which is composed of axon bundles of ganglion cells [7]. The number of CB immunoreactive neurons decreased more than that of CR immunoreactive neurons in both ischemic retinas when compared with the number of CB and CR immunoreactive neurons in the GCL. These findings are partly inconsistent with another report [10], which documented that CB immunoreactive cells in the GCL of the rabbit retina are less reduced and, therefore, are more vulnerable than CR immunoreactive neurons under the pressure-induced ischemic condition. The number of CR immunoreactive neurons in the GCL decreased more than that of the CB neurons, but CR immunoreactivity became stronger in the axon bundles of the NFL in both ischemic retinas, suggesting that CR in the ganglion cell axons are distributed in a membrane or structure bound manner. This suggestion was confirmed by Mojumder et al. [21] who reported that CB and CR are expressed in the cell soma of the ganglion cells and that CR is concentrated in the axonal regions, which is most likely membrane bound.
CR immunoreactivity in the axon bundles appeared stronger in the MCAO ischemic retinas than that in the EIOP ischemic retinas. It is generally accepted that calcium binding proteins play a role as Ca2+ buffers, as they are determinants of intracellular Ca2+ fluctuation kinetics [23]. Considering the role of calcium binding proteins, the significance of this finding infers that CR in the MCAO ischemic retinas was concentrated in the axonal regions due to excess Ca2+ buffering against ischemic damage by the blood flow blockade. In contrast, CB expression increases in transient forebrain ischemia and spinal ischemia [24, 25]. An increase in CB expression under the ischemic condition in these regions may be associated with resistance to neuronal degeneration. This hypothesis was confirmed by other reports [26-28]. Although a direct comparison of results is difficult because of differences in animal species and target organs, combining our findings with those, the MCAO ischemia effect was initiated from the axon of the ganglion cells, so CR expression increased in the axon bundles for excess Ca2+ buffering.
SMI32 immunoreactivity appeared in the NFL axon bundles in normal retinas and even in the cell soma of some ganglion cells in MCAO ischemic retinas. The SMI32 antibody recognizes a dephosphorylated epitope on the carboxyl-terminal regions of the NF-M and NF-H subunits [29]. SMI32 immunoreactivity appears in the cell soma and cell processes of large ganglion cells and amacrine cells in the mouse retina [30, 31] and in the axons of rat and monkey ganglion cells [32, 33]. This discrepancy may originate from the antibody source, epitopes, or animal species differences. SMI32 immunoreactivity has been investigated in the axotomized rat retina [32], and in the monkey chronic glaucoma retina [33]. In these studies, dephosphorylated rather than phosphorylated neurofilaments occurred in degenerating nerve fibers of the control. In these reports, they concluded that dephosphorylation of neurofilaments is involved in ischemic damage to axonal transport and to further Wallerian degeneration. When we compared these results with our findings, the transient ischemic damage in the MCAO model may have been caused by an axonal transport disturbance by stimulating NF dephosphorylation in the NFL ganglion axons.
GFAP immunoreactivity is normally detected in the astrocytes and end feet of the Müller cells in rat retinas. In the present study, GFAP immunoreactivity appeared in the entire profile of Müller glial cells in EIOP ischemic retinas and was limited to the astrocytes and the proximal end of Müller glial cells in MCAO ischemic retinas. In response to dangerous stimuli, Müller glial cells of the retina are activated with upregulated expression of the stress filament protein GFAP, in addition to the ordinary intermediate filament protein vimentin [2, 4, 34]. As in our study, Müller glial cells showed strong GFAP immunoreactivity in a time-dependent manner in a pressure-induced ischemia model of the rat retina [4]. In contrast, loss of astrocytic GFAP staining has been reported in the optic nerve head of a chronic glaucoma model made by limbal vein cauterization [2]. In contrast to our results, GFAP immunoreactivity also appears in Müller cells in MCAO model retinas [34]. Taken together, the Müller glial reaction following GFAP labeling indicates that it occurred in response to a kind of mechanical stimuli compression by an excessively raised IOP. Bringmann et al. [35] have reviewed the neuroprotective and detrimental effects of Müller cell gliosis and summarized that reactive Müller glial cells protect tissue from further damage and preserve tissue function by releasing antioxidants and neurotrophic factors; however, Müller cell gliosis can also contribute to neuronal degeneration. Based on this review [35] and reports on neuronal death in ischemic retinas [7, 11, 27, 36], our glial reaction results suggest that Müller gliosis is detrimental to neuronal degeneration.
In conclusion, our results suggest that EIOP ischemic retinas developed more severe neuronal degeneration due to a particularly vigorous glial reaction by mechanical stress stimuli, whereas MCAO ischemic retinas presented with comparatively mild neuronal degeneration triggered only by an axonal transport disturbance.
Figures and Tables
Fig. 1
Light micrographs showing immunohistochemical changes in the retina taken from normal (A), 3 days (B) and 14 days (C) after elevation of intraocular pressure, and 1 day (D), 3 days (E), and 7 days (F) after middle cerebral artery occlusion, which were processed for anti-calbindin immunohistochemistry. ONL, outer nuclear layer; INL, inner nuclear layer; GCL, ganglion cell layer. Scale bar=50 µm.
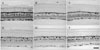
Fig. 2
Quantitative analysis of the number of calbindin D28K-immunoreactive neurons in the ganglion cell layer in the retinas at normal (N) and at 1 day (1D), 3 days (3D), 7 days (7D), and 14 days (14D) after both elevation of intraocular pressure (EIOP) and middle cerebral artery occlusion (MCAO), which is represented as mean cell number and standard deviation appearing per 1 mm length of 5 µm-thick vertical retinal section. *P<0.05; Student's t-test.
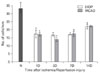
Fig. 3
Light micrographs showing immunohistochemical changes in the retina taken from normal (A), 1 day (B) and 14 days (C) after elevation of intraocular pressure, and 3 days (D), 7 days (E), and 14 days (F) after middle cerebral artery occlusion, which were processed for anti-calretinin immunohistochemistry. ONL, outer nuclear layer; INL, inner nuclear layer; GCL, ganglion cell layer. Scale bar=50 µm.
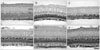
Fig. 4
Quantitative analysis of the number of calretinin-immunoreactive neurons in the ganglion cell layer in the retinas at normal (N) and at 1 day (1D), 3 days (3D), 7 days (7D), and 14 days (14D) after both elevation of intraocular pressure (EIOP) and middle cerebral artery occlusion (MCAO). Data represented as mean cell number and standard deviation appearing per 1 mm length of 5 µm-thick vertical retinal section. *P<0.05; Student's t-test.
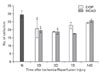
Fig. 5
Light micrographs showing immunohistochemical changes in the retina taken from 3 days (A) and 7 days (B) after elevation of intraocular pressure, and 3 days (C) and 7 days (D) after middle cerebral artery occlusion, which were processed for anti-SMI32 immunohistochemistry. Arrows indicate SMI-immunoreactive ganglion cells in the ganglion cell layer. ONL, outer nuclear layer; INL, inner nuclear layer; IPL, inner plexiform layer. Scale bar=50 µm.
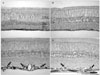
Fig. 6
Densitometric analyses of immunoblots of calbindin D28K (A), calretinin (B), and SMI32 (C) protein levels in the retinas at normal (N) and at 1 day (1D), 3 days (3D), 7 days (7D), and 14 days (14D) after both elevation of intraocular pressure (EIOP) and middle cerebral artery occlusion (MCAO). Data are shown as means±standard deviation. *P<0.05, Student's t-test.
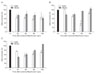
Fig. 7
Light micrographs showing immunohistochemical changes in the retina taken from normal (A), 7 days (B) after elevation of intraocular pressure, and 3 days (C) and 7 days (D) after middle cerebral artery occlusion, which were processed for anti-glial fibrillary acidic protein immunohistochemistry. ONL, outer nuclear layer; INL, inner nuclear layer; IPL, inner plexiform layer; GCL, ganglion cell layer. Scale bar=50 µm.
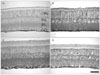
Acknowledgements
This study was supported by the Ministry of Education, Science and Technology in Republic of Korea (2010-0002120).
References
1. Sommer A. Intraocular pressure and glaucoma. Am J Ophthalmol. 1989. 107:186–188.
2. Johnson EC, Deppmeier LM, Wentzien SK, Hsu I, Morrison JC. Chronology of optic nerve head and retinal responses to elevated intraocular pressure. Invest Ophthalmol Vis Sci. 2000. 41:431–442.
3. Johnson EC, Morrison JC. Friend or foe? Resolving the impact of glial responses in glaucoma. J Glaucoma. 2009. 18:341–353.
4. Kim IB, Kim KY, Joo CK, Lee MY, Oh SJ, Chung JW, Chun MH. Reaction of Muller cells after increased intraocular pressure in the rat retina. Exp Brain Res. 1998. 121:419–424.
5. Lewis GP, Fisher SK. Up-regulation of glial fibrillary acidic protein in response to retinal injury: its potential role in glial remodeling and a comparison to vimentin expression. Int Rev Cytol. 2003. 230:263–290.
6. Büchi ER. Cell death in the rat retina after a pressure-induced ischaemia-reperfusion insult: an electron microscopic study. I. Ganglion cell layer and inner nuclear layer. Exp Eye Res. 1992. 55:605–613.
7. Ahmed FA, Chaudhary P, Sharma SC. Effects of increased intraocular pressure on rat retinal ganglion cells. Int J Dev Neurosci. 2001. 19:209–218.
8. Dijk F, Kamphuis W. An immunocytochemical study on specific amacrine cell subpopulations in the rat retina after ischemia. Brain Res. 2004. 1026:205–217.
9. Dijk F, van Leeuwen S, Kamphuis W. Differential effects of ischemia/reperfusion on amacrine cell subtype-specific transcript levels in the rat retina. Brain Res. 2004. 1026:194–204.
10. Kwon OJ, Kim JY, Kim SY, Jeon CJ. Alterations in the localization of calbindin D28K-, calretinin-, and parvalbumin-immunoreactive neurons of rabbit retinal ganglion cell layer from ischemia and reperfusion. Mol Cells. 2005. 19:382–390.
11. Du Y, Hirooka K, Miyamoto O, Itano T, Tokuda M, Shiraga F. Both amacrine and bipolar cells release glutamate in the rat retina after ischemia/reperfusion insult in vitro. Curr Eye Res. 2008. 33:782–788.
12. Sucher NJ, Lipton SA, Dreyer EB. Molecular basis of glutamate toxicity in retinal ganglion cells. Vision Res. 1997. 37:3483–3493.
13. Naskar R, Dreyer EB. New horizons in neuroprotection. Surv Ophthalmol. 2001. 45:Suppl 3. S250–S255.
14. Ullian EM, Barkis WB, Chen S, Diamond JS, Barres BA. Invulnerability of retinal ganglion cells to NMDA excitotoxicity. Mol Cell Neurosci. 2004. 26:544–557.
15. Osborne NN, Ugarte M, Chao M, Chidlow G, Bae JH, Wood JP, Nash MS. Neuroprotection in relation to retinal ischemia and relevance to glaucoma. Surv Ophthalmol. 1999. 43:Suppl 1. S102–S128.
16. Weinreb RN, Khaw PT. Primary open-angle glaucoma. Lancet. 2004. 363:1711–1720.
17. Longa EZ, Weinstein PR, Carlson S, Cummins R. Reversible middle cerebral artery occlusion without craniectomy in rats. Stroke. 1989. 20:84–91.
18. Lowry OH, Rosenbrough NJ, Farr AL, Randall RJ. Protein measurement with the Folin phenol reagent. J Biol Chem. 1951. 193:265–275.
19. Peterson GL. Review of the Folin phenol protein quantitation method of Lowry, Rosenbrough, Farr and Randall. Anal Biochem. 1979. 100:201–220.
20. Rogers JH. Calretinin: a gene for a novel calcium-binding protein expressed principally in neurons. J Cell Biol. 1987. 105:1343–1353.
21. Mojumder DK, Wensel TG, Frishman LJ. Subcellular compartmentalization of two calcium binding proteins, calretinin and calbindin-28 kDa, in ganglion and amacrine cells of the rat retina. Mol Vis. 2008. 14:1600–1613.
22. Kim SA, Jeon JH, Son MJ, Cha J, Chun MH, Kim IB. Changes in transcript and protein levels of calbindin D28k, calretinin and parvalbumin, and numbers of neuronal populations expressing these proteins in an ischemia model of rat retina. Anat Cell Biol. 2010. 43:218–229.
23. Baimbridge KG, Celio MR, Rogers JH. Calcium-binding proteins in the nervous system. Trends Neurosci. 1992. 15:303–308.
24. Hwang IK, Kang TC, Lee JC, Park SK, An SJ, Lee IS, Lee YB, Sohn HS, Kang JH, Choi SY, Won MH. Chronological alterations of calbindin D-28k immunoreactivity in the gerbil main olfactory bulb after ischemic insult. Brain Res. 2003. 971:250–254.
25. Lee JC, Hwang IK, Yoo KY, Jung JY, Cho JH, Moon SM, Kang TC, Kim WK, Kim YS, Won MH. Calbindin D-28k is expressed in the microvascular basal lamina in the ventral horn at early time after transient spinal cord ischemia in the rabbit. Brain Res. 2005. 1047:123–128.
26. D'Orlando C, Fellay B, Schwaller B, Salicio V, Bloc A, Gotzos V, Celio MR. Calretinin and calbindin D-28k delay the onset of cell death after excitotoxic stimulation in transfected P19 cells. Brain Res. 2001. 909:145–158.
27. Wang X, Ng YK, Tay SS. Factors contributing to neuronal degeneration in retinas of experimental glaucomatous rats. J Neurosci Res. 2005. 82:674–689.
28. Fan Y, Shi L, Gu Y, Zhao Y, Xie J, Qiao J, Yang GY, Wang Y, Lu CZ. Pretreatment with PTD-calbindin D 28k alleviates rat brain injury induced by ischemia and reperfusion. J Cereb Blood Flow Metab. 2007. 27:719–728.
29. Soifer D, Nicoletti V, Cabane K, Mack K, Poulos B. Expression of the neurofilament protein NF-H in L cells. J Neurosci Res. 1991. 30:63–71.
30. Lim EJ, Kim IB, Oh SJ, Chun MH. Identification and characterization of SMI32-immunoreactive amacrine cells in the mouse retina. Neurosci Lett. 2007. 424:199–202.
31. Lin H, Zhai J, Cañete-Soler R, Schlaepfer WW. 3' untranslated region in a light neurofilament (NF-L) mRNA triggers aggregation of NF-L and mutant superoxide dismutase 1 proteins in neuronal cells. J Neurosci. 2004. 24:2716–2726.
32. Meller D, Eysel UT, Schmidt-Kastner R. Transient immunohistochemical labelling of rat retinal axons during Wallerian degeneration by a monoclonal antibody to neurofilaments. Brain Res. 1994. 648:162–166.
33. Kashiwagi K, Ou B, Nakamura S, Tanaka Y, Suzuki M, Tsukahara S. Increase in dephosphorylation of the heavy neurofilament subunit in the monkey chronic glaucoma model. Invest Ophthalmol Vis Sci. 2003. 44:154–159.
34. Kalesnykas G, Tuulos T, Uusitalo H, Jolkkonen J. Neurodegeneration and cellular stress in the retina and optic nerve in rat cerebral ischemia and hypoperfusion models. Neuroscience. 2008. 155:937–947.
35. Bringmann A, Iandiev I, Pannicke T, Wurm A, Hollborn M, Wiedemann P, Osborne NN, Reichenbach A. Cellular signaling and factors involved in Muller cell gliosis: neuroprotective and detrimental effects. Prog Retin Eye Res. 2009. 28:423–451.
36. Steele EC Jr, Guo Q, Namura S. Filamentous middle cerebral artery occlusion causes ischemic damage to the retina in mice. Stroke. 2008. 39:2099–2104.