Abstract
During the nervous system development, immature neuroblasts have a strong potential to migrate toward their destination. In the adult brain, new neurons are continuously generated in the neurogenic niche located near the ventricle, and the newly generated cells actively migrate toward their destination, olfactory bulb, via highly specialized migratory route called rostral migratory stream (RMS). Neuroblasts in the RMS form chains by their homophilic interactions, and the neuroblasts in chains continually migrate through the tunnels formed by meshwork of astrocytes, glial tube. This review focuses on the development and structure of RMS and the regulation of neuroblast migration in the RMS. Better understanding of RMS migration may be crucial for improving functional replacement therapy by supplying endogenous neuronal cells to the injury sites more efficiently.
Interneurons in the olfactory bulb (OB) are produced from the lateral ganglionic eminences (LGE) during embryonic development and from the anterior subventricular zone (SVZa) in the lateral ventricles postnatally. Upon genesis from neural stem cells, neuronal precursors then migrate tangentially to the OB through a distinct pathway called the rostral migratory stream (RMS). Within the OB, neuroblasts finally locate in the granule cell layer and glomerular layer and differentiate into the granule and periglomerular cells, respectively. This migration occurs throughout life in many species including rodents, primates, and humans (Lois & Alvarez-Buylla, 1994; Kornack & Rakic, 2001; Curtis et al., 2007). It is known that migrating neuroblasts form chain-like homophilic association, and they migrate tangentially without the aid of radial fibers (Lois et al., 1996). This is one of the longest migrations in CNS development, and the speed of chain migration (70~80 µm/hr) is considerably faster than other types of migration (Nam et al., 2007).
In addition to migrating neuroblasts, RMS contains specialized surrounding glial cells, which form tube-like structure (glial tube), serving as a scaffold of migration neuroblasts. The migration of neuronal precursors into the OB is regulated by the orchestrated activities of multiple secretory signals, such as repulsive factors in the septum, motogenic factors in the SVZa and the RMS, as well as chemoattractive factors derived from the OB or olfactory epithelium (Coskun & Luskin, 2002; Whitman & Greer, 2009). Furthermore, cell-cell adhesions and extracellular matrix proteins also significantly affect the mode, speed and direction of neuroblasts. In this review, we will overview development and anatomy of RMS and regulatory signals for neuronal migration in the RMS.
RMS is organized as a tubular extension of the lateral ventricle reaching the OB. Rodent RMS arises from the SVZ of the lateral ventricle, proceeds to the rostrodorsal aspect of the striatum to the rostroventral aspect of the striatum and then extends rostrally into the olfactory tract (Fig. 1). The RMS tract terminates in the subependymal layer, the central region of OB granule cell layer (Lois & Alvarez-Buylla, 1994; Lois et al., 1996). Initial formation of RMS occurs during the embryonic development on E15-17 in mouse (Pencea & Luskin, 2003). Generation of RMS precedes the bulge of the OB and formation of the olfactory ventricle which is an extension of anterior lateral ventricle. As the OB and ventricle begin to form, the developing RMS lies laterally from the olfactory ventricle. In primates and humans, the RMS tube is very narrow and forms a sigmoidally curved path to laterally circumscribe the striatum (Curtis et al., 2007). Although the morphology and structure of rodent vs. human RMS are different, RMS in humans also contains migrating neuroblasts toward the olfactory tubercle, and the OB neurogenesis appears to persist in adulthood (Bédard & Parent, 2004).
RMS is composed of different cell types. Most common cells found in the RMS are migrating neuroblasts. After the birth of neuroblasts in the SVZ, they mutually interact and organize themselves into chains. Although migration of neuroblasts in RMS is mostly toward the rostral part, live imaging of migrating neuroblasts demonstrates that their movement is rather complex, and they can stop or move reverse direction (Nam et al., 2007). Chain formation appears to be constraint for the migrating neuroblasts to the rostral direction. It is known that neuroblasts in the RMS form chain-like associations with gap or adherens junctions with other neuroblasts (Miragall et al., 1997; Marins et al., 2009), so that these cells can migrate step on other neuroblasts as 'chains'.
Neural cell adhesion molecule (NCAM) and its modification, polysialylated-NCAM (PSA-NCAM), appear to be critical for RMS migration. Removal of PSA from PSA-NCAM results in the dissociation of neuroblast chains. Under this condition, neuroblasts fail to migrate in the RMS, and they start to scatter into the striatum or corpus callosum, supporting the importance of chain formation for RMS directional migration (Battista & Rutishauser, 2010). Furthermore, it is known that neuronal migration occurs in community, and thus the chimeric mosaics of normal neuroblasts with migration-defected neuroblasts in the developing cerebellum partially rescues the migration defect. This suggests that neuroblasts migrate in clusters and this 'community' plays a significant role (Yang et al., 2002). Consistently, transplantation of mesenchimal stem cells into RMS results in the directional migration of these heterologous stem cells. Conversely, transplantated SVZ neural stem cells in the striatum do not form chain. Instead, they randomly disperse, supporting the idea that chain structure is critical for the directional migration of SVZ-derived neuroblasts (Zigova et al., 1998; Yamashita et al., 2006).
In the RMS, specialized astrocytes form glial tubes and surround migrating neuroblasts. Normally, gliogenesis is slower than neurogenesis in many regions during brain development, and the RMS glial tube forms postnatally (Law et al., 1999). Therefore, from the embryonic to early postnatal stage, neuroblasts migrate through the RMS without glial tube, suggesting that migration of neonatal SVZ-derived cells to the OB does not require glial cells, which is in contrast to the radial migration of immature, newly postmitotic CNS neurons in the developing telencephalon (Hatten 1999). In the adult brain, the glial tube contributes to the regulation of RMS migration and when disrupted, the development severely impairs RMS migration. Deletion of the Vax1 homeobox gene leads to a severe disruption of the RMS glial tube formation, resting in the small OB (Soria et al., 2004). Similarly, the formation of glial tubes is disrupted in Bax-deficient mice, presumably due to the outflow of migrating neuroblasts. In this mutant, RMS migration is progressively impaired, and no addition of new cells in the adult OB although their embryonic/postnatal migration was normal (Kim et al., 2007). This result also suggests that RMS glial tube is especially important for RMS migration in the adult brain.
Most astrocytes in the forebrain are produced from Olig2-expressing neural stem cells. Although same Olig2-expressing neural stem cells produce both neuroblasts and forebrain astrocytes, neuroblasts take RMS tangential migratory route, and astrocytes migrate radially to become forebrain astrocytes (Zhang 2001; Marshall et al., 2005; Ono et al., 2009). Currently it is yet unclear whether astrocytes forming glial tube are also produced from Olig2-expressing stem cells. It appears that the interactions between astrocytes and migrating neuroblasts instruct glial tube formation. It has been known that neuroblasts do not form proper chains in beta1 integrin-deficient mice, and they show the disruption of glial tubes, suggesting that chains of migration neuroblasts may be necessary for maintenance of glial tube (Belvindrah et al., 2007). Recently it has been found that migrating neuroblasts can modulate the structure of glial tube and have the ability to remove the impeding astrocytic processes away from their migrating route. This ability of neuroblasts may constantly modify the organization of the meshwork of the glial tube, helping efficient migration of neuroblast chains through the compact and narrow glial channels without creating choke points along the RMS (Eom et al., 2010; Kaneko et al., 2010).
Recent studies have also revealed that blood vasculature within the RMS is arranged in parallel with migration route, and this specialized arrangement of vessels provides additional scaffolds for neuronal chain migration (Snapyan et al., 2009; Whitman et al., 2009). Migrating neuroblasts directly contact to blood vessels, and vascular endothelial cells release BDNF which is a modulator for RMS migration. Glial cells are also intimately associated with blood vessels, and complex communications among neuroblasts-astrocyte-vessels may be required for proper RMS migration. As mentioned above, development of astrocytes occurs during the postnatal development. In addition, parallel alignment of blood vessels in the RMS is not yet established in the embryonic RMS, suggesting that the migration of neuroblasts in the embryonic/early postnatal stages and in adulthood may be instructively different in terms of the contribution of glial and vascular cells.
Although most neural stem cells reside in the SVZ, multipotent neural precursors are also found in the RMS and OB (Pencea et al., 2001; Gritti et al., 2002; Liu & Martin, 2003; Giachino & Taylor, 2009). Because neural stem cells in the RMS maintain multiple potency, they can produce oligodendrocytes as well as neurons and astrocytes in vitro or in response to brain injury (Gritti et al., 2002; Gotts & Chesselet, 2005; Cayre et al., 2006; Jablonska et al., 2010). In fact, it has been known that neural stem cells in the SVZ are highly heterogeneous, and neural stem cells in different anatomical regions exhibit different ability to produce specific types of neuronal cell populations (Merkle et al., 2007). Likewise, neural stem cells in RMS and OB are different from SVZ stem cells in their differentiation potential. For instance, calretinin-expressing GABAergic interneurons destined to the olfactory granule cell layers are mainly generated from the SVZ stem cells whereas perigloerular dopaminergic neurons are produced from the RMS. Because these differences are mirrored by different expression of transcription factors such as Olig2 and Pax6, these results suggest that intrinsic mechanisms are involved in the differentiation of specific neuronal populations (Hack et al., 2005; Lledo et al., 2008).
RMS migration is regulated by multiple factors in multiple steps (Fig. 2). Newly specified neuroblasts form chains to initiate RMS migration (Step 1), and they directionally move toward the OB (Step 2). During this chain migration, many factors influence the speed and direction of the migration. These factors include contact information from cell-cell adhesion/extracellular matrix (ECM), chemoattractive or chemorepulsive secretory factors, and local influence from blood vessels and glial tube. Upon arrival at the OB, neuroblasts detach from the chains, and they initiate radial gila-dependent migration within the OB (Step 3). The journey of neuroblasts from the SVZ to OB is terminated by detachment of them from radial migration.
Migrating neuroblasts are born from specialized cellular niche in the SVZ. In this niche, neural stem cells (type B cells) which exhibit astrocyte-like phenotypes slowly proliferate and produce type C transit-amplifying cells. These transit-amplifying cells form clusters within this niche and they rapidly generate numerous type A migrating neuroblasts (Doetsch 2003). Therefore, right after their birth, neuroblasts can associate with other newly produced cells as large clusters. Indeed, homophilic NCAM express from the transit-amplifying cell stages, which ensue the cluster formation of them and their progenitor cells (neuroblasts). Therefore, it appears that chain formation by homophilic aggregation of migrating neuroblasts is a spontaneous and autonomous process.
Within the SVZ, the flow of cerebral spinal fluid (CSF) may influence the initiation of the migratory process. Ventriclular walls are covered by ciliated ependymal cells, and the synchronized beating of ependymal cilia creates a caudal to rostral flow of CSF. In mutant mice with defective cilia, CSF flow is greatly perturbed, and the neuroblasts fail to migrate properly from the SVZ into the RMS (Sawamoto et al., 2006). It is further demonstrated that failure of the gradient formation of chemorepulsive signals in this mutant mice is responsible for the migration-defective phenotype.
Cell-cell adhesion and extracellular matrix: Homophilic interaction of neuroblasts is essential for the maintenance of migratory chains, as mentioned above. NCAM is a major cell adhesion molecule expressed in the migrating neuroblasts. An essential role of NCAM for the RMS migration is decisively seen in NCAM-deficient mice which exhibit the small OB and cumulation of neuroblasts in the caudal pole of the RMS (Cremer et al., 1994; Hu et al., 1996). Migratory failure was especially profound during the embryonic development, suggesting that NCAM-dependent chain formation is critical for the embryonic/early postnatal stage of RMS migration before the glial tube/vasculature maturation. NCAM in RMS neuroblasts are highly polysialylated (Hu et al., 1996). Removal of PSA from NCAM causes dispersion of chains both in vivo and in vitro (Chazal et al., 2000; Battista & Rutishauser, 2010). Whereas the NCAM deficiency in mice results in a failure of RMS migration, removal of PSA from NCAM promotes an ectopic migration of neuroblasts to the surrounding brain regions, including the cerebral cortex and striatum. These observations suggest that polysialylation plays a critical role in the maintenance of neuroblasts in chains, as opposed to the NCAM is required for the migration/movement of neuroblasts. In addition, a recent report also demonstrated that NCAM could induce proliferation of SVZ neural stem cells without PSA modification (Boutin et al., 2009).
ECM proteins are essential for the RMS migration. Matrix metalloproteases (MMPs) are zinc-dependent proteases with ECM modifying activities, and best known for their roles in the tumor invasion/metastasis (Kessenbrock et al., 2010). RMS expresses MMPs, and MMP activity is required for the migration of single neuroblasts (Bovetti et al., 2007). Interestingly, suppression of MMP activity does not interfere with chain migration, suggesting that MMP-related ECM remodeling is related to the attachment/detachment of neuroblasts in chains. Integrin α6β1 is also expressed in migrating neuroblasts in mature RMS pathways and they interact with laminins in the extracellular matrix to guide the migration (Emsley & Hagg, 2003). Blockade of integrin signaling perturbs chain and promots dispersion of migrating cells (Murase & Horwitz, 2002; Emsley & Hagg, 2003). Integrin-dependent regulation of RMS migration is at least in part dependent on the other chemoattracting signals, Netrins and deleted in colorectal cancer (DCC) (Murase & Horwitz, 2002; Staquicini et al., 2009). ADAM, a disintegrin and metalloprotease, is a multidomain protein whith metalloprotease and disintegrin domains. ADAM2 is expressed in the RMS neuroblasts, and ADAM2-deficient mice exhibit perturbation of RMS migration (Murase et al., 2008).
Chemoattractive and chemorepulsive signals: Many soluble factors utilized for chemoattaractive or repulsive signals for axon guidance are also involved in the regulation of RMS migration. For instance, Netrin, serving for chemoattactants during development, is expressed by mitral cells in the OB and RMS astrocytes, and its receptor, DCC, is expressed in the migrating neuroblasts. Blocking DCC signaling alters the direction of protrusions on migrating cells, indicating the significance of Netrin-DCC signaling in the RMS migration (Murase & Horwitz, 2002). Ephrin-Eph signalings are also implicated in the RMS migration although this effect may be mediated indirectly by the regulation of SVZ cell proliferation (Conover et al., 2000).
Repulsive signals for axon guidance are involved in the directional RMS migration as a repellant (Nguyen-Ba-Charvet et al., 2004). Chemorepulsive Slit proteins are secreted from the posterior brain structures such as the septum, and Robos known as their receptors are expressed in the SVZ and RMS neuroblasts. In the absence of Slit, a subset of neuroblasts migrates caudally, suggesting that Slit-Robo signaling acts as a repulsive signal. In addition, Slit is released from neuroblasts themselves and modulates astrocyte networks in the RMS (Eom et al., 2010; Kaneko et al., 2010).
Many growth/neurotrophic factors are identified as chemoattractive factors for RMS migration. These factors include glial cell line-derived neurotrophic factor (GDNF), hepatocyte growth factor (HGF), insulin-like growth factor (IGF-1), vascular endothelial growth factor (VEGF) and brain-derived neurotrophic factor (BDNF) (Paratcha et al., 2006; Chiaramello et al., 2007; Garzotto et al., 2008; Hurtado-Chong et al., 2009; Wittko et al., 2009). GDNF activates cdk5, which is a critical intracellular signaling molecule for the radial migration of neuroblasts during development. In addition, the function of GDNF is dependent on the direct interaction of GDNF on NCAM (Paratcha et al., 2006). HGF also called as a scatter factor has been originally identified as a regeneration-promoting factor in the liver and a motogen for epithelial cells. Now it is considered as a pleiotrophic factor with neurotrophic activities (Maina & Klein, 1999; Sun et al., 2002b). HGF and its receptor c-Met are involved in the radial migration of cortical neurons and tangential migration of GABAergic interneurons during the brain development (Powell et al., 2001; Sun et al., 2002a). Involvement of HGF in the RMS migration is recently identified, and Ras/MAPK downstream signaling is activated by HGF activation via interaction of cMet with Grb2 in the RMS (Garzotto et al., 2008). Although HGF functions as a motogen and does not promote directional migration in many cell types, it appears that HGF acts as a chemoattractant and involves in the directional migration of neuroblasts in the RMS. IGF-1 is a growth factor found in the developing brain, and its expression levels decrease postnatally in most brain reigons except the OB (Russo et al., 2005). The exit of neurobalsts from the SVZ to RMS is impaired and the neuroblasts are accumulated in SVZ in IGF-1 deficient mice. Migration promoting effect of IGF-1 is mediated by PI3K- and Src family kinase (SFK) signal cascades (Hurtado-Chong et al., 2009). VEGF is a major regulator of vasculogenesis and angiogenesis (Olsson et al., 2006). VEGF is also expressed within SVZ and RMS glial cells (Balenci et al., 2007). Deficiency of VEGFR-1 signaling induces the alternations in migration speed along the RMS (Wittko et al., 2009). BDNF and its receptor TrkB are expressed in the adult RMS-OB (Mackay-Sima & Chuahb, 2000). BDNF is suggested as a chemoattractant for SVZ neuroblasts migration (Chiaramello et al., 2007).
Sonic hedgehog (Shh) signaling is important for early developmental processes, and directly acts on the proliferation of SVZ stem cells. Unexpectedly, Shh signals also function as a regulator of RMS migration indirectly (Balordi & Fishell, 2007; Angot et al., 2008). Absence of Shh signals promotes precocious differentiation of neural stem cells/transit-amplifying cells, resulting in the transient increase in the pool of migrating neuroblasts. However, these cells fail to express Slit ligand, and they fail to migrate properly.
Long-distance cues from the OB are believed to be less important for the RMS migration. This idea was supported by observations that RMS migrations were normal after surgical removal of the OB (Kirschenbaum et al., 1999). Similarly, lesions disconnecting the RMS from the OB also do not prevent RMS migration, and neuroblasts accumulate at the point of lesion (Jankovski et al., 1998). However, these results should be carefully interpreted because brain injury is known to recruit neuroblasts to the injured sites (Lee et al., 2006; Li et al., 2008). Because these invasive methods should produce injury on their migratory routes, injury-induced chemoattractants may compensate for the absence of endogenous OB-derived signals (Liu & Rao, 2003). Therefore, it is required to be re-examined whether there are long-distance chemoattractive cues released from the OB.
Local influence within RMS: As we discussed earlier, local interactions between migrating neuroblasts and other cell types in the RMS (e.g. astrocytes and blood vessels) are important for the control of RMS migration. In addition to their scaffolding activities, astrocytes in the RMS can modulate the speed of migrating neuroblasts via modification of GABA signaling. Astrocytes express the GABA transporter GAT4, and they rapidly remove extracellular GABA concentration. Extracellular GABA reduces migratory speed, and astrocytes modulate RMS migration through GAT4 activity (Bolteus & Bordey, 2004; Platel et al., 2008). RMS astrocytes are sources of secretory factors for the modulation of RMS migration, including Netrin and VEGF (Mason et al., 2001; Murase & Horwitz, 2002; Balenci et al., 2007; Staquicini et al., 2009).
Blood vessels also function as a source for secretory molecules in addition to their scaffolding function. Blood vessels release BDNF which triggers low-affinity p75 receptor activation in the neuroblasts (Snapyan et al., 2009). Furthermore, since high-affinity BDNF receptor TrkB is expressed in the glial tube, competition of neuroblasts and astrocytes for BDNF appears to play a modulatory role in the RMS migration.
When the neuroblasts arrive at the OB, they detach from the chains and migrate radially to their final positions, the granule cell layer or glomerular layer. Conditional deletion of the receptor tyrosine kinase ErbB4, which interacts with its ligands, neuregulins, in mouse neural stem cells/neuroblasts resulted in the accumulation of cells in the subependymal layer (which is outlet of RMS) within the OB. This result suggests that ErbB4 signaling is involved in the switching from tangential to radial migration (Anton et al., 2004). Extracellular glycoprotein, Tenascin-R, plays unique role in the switching of neuroblasts from RMS migration to radial migration (Saghatelyan et al., 2004). Tenascin-R is enriched in the OB, and their expression is dependent on OB neuronal activity. Ectopic expression of Tenascin-R in non-neurogenic regions can trigger neuroblasts to dissociate from their chain and migrate toward Tenascin-expressing ectopic location, suggesting that Tenascin-R mediates the activity-dependent transition of chain migration to radial migration in the OB.
Reelin is a large, secretory glycoprotein which has been recognized as a critical regulator of radial migration during embryonic development (Rice & Curran, 2001). Receptors for Reelin are apolipoprotein E receptor 2 (ApoER2) and very low-density lipoprotein receptor (VLDLR). Reelin signal is propagated intracellularly via phosphorylation of adaptor protein disabled-1 (Dab1). In addition to well-documented role of Reelin in the radial migration, Reelin signaling also acts as a detachment signal in RMS chain migration (Hack et al., 2002; Kim et al., 2002). Reelin is expressed from Mitral cells in the OB, and ApoER2 and Dab1 are expressed in the migrating RMS neuroblasts. Neuronal progenitors fail to maintain in chains in response to exogenousely applied Reelin protein in vitro, and Reeler mutant mice exhibit ectopic accumulation of neuroblasts in the RMS, failing to transit from RMS migration to radial migration. Intracellular signaling cascade in the migrating neuroblasts includes SFK and Erk signal activation (Simό et al., 2007). Finally, ApoER2 and VLDLR signalings are activated in the RMS not only by Reelin but also a novel ligand, thrombospondin-1 (Andrade et al., 2007; Blake et al., 2008).
In the OB, neuroblasts differentiate into either GABAergic neurons in the olfactory granule cell layer, or dopaminergic neurons in the periglomeruli. Different subsets of neuroblasts begin to express multiple transcription factors such as Pax6, Emx1, Dlx5/6, Sp8, Gsx2 and Er81 in the OB, which promote granule cell layer GABAergic and periglomerular dopaminergic neuronal differentiation (Hack et al., 2005; Kohwi et al., 2005; Kohwi et al., 2007; Saino-Saito et al., 2007; Waclaw et al., 2009). Differentiation of neuroblasts in the OB is multi-step processes. Newly produced granule cells exhibit dendritic arbors and first signs of synaptic connections (advents of dendritic spines and spontaneous action potentials) are recognized within 2~3 weeks after production (Petreanu & Alvarez-Buylla, 2002; Mizrahi 2007; Grubb et al., 2008). Recent study has proposed that circuit integration of adult-generated OB neurons is much quicker, and functional GABAergic input to newly migrated neurons is first seen as early as 3 days after their OB arrival (Panzanelli et al., 2009).
During the differentiation/maturation of newly generated OB neurons, approximately 30~70% of them does not survive and undergoes programmed cell death (PCD) (Petreanu & Alvarez-Buylla, 2002; Winner et al., 2002; Kim et al., 2007). PCD occurs during the synaptogenesis period when neurons are integrating into the olfactory circuits (Yamaguchi & Mori, 2005). During development, PCD mainly occurs during the synaptogenesis period, which serves as a means to numerically match the input neurons to target for efficient systems-matching (Buss et al., 2006). Therefore, the extent of demand for synaptic activity/plasticity may be a critical factor for the regulation of PCD. In consistent, the conditions which increase OB circuit activity, such as enriched odor exposure or olfactory learning, increase survival (Rochefort et al., 2002; Alonso et al., 2006; Mouret et al., 2008). Especially, not simple olfaction, but olfactory activity associated with behavioral tasks appears to play more important roles in the survival. Conversely, reduction of OB activity by naris closure or destruction of olfactory epithelium decreases the surviving neuronal number (Mandairon et al., 2003; Saghatelyan et al., 2005; Mandairon et al., 2006).
Biochemically, PCD of adult-produced OB neurons is caspase-dependent apoptosis. Recently, we have examined the contribution of pro-apoptotic molecule Bax on this process (Kim et al., 2007). In the Bax-deficient (Bax-KO) mice, caspase-3 activation was virtually absent, and PCD of adult-produced neuroblasts was completely prevented. Interestingly, Bax-KO mice exhibited failure in the RMS migration as well as absence of PCD in the OB. Careful examination of RMS development revealed that failure of glial tube formation may be responsible for the migration defect in Bax-KO mice. In addition to the OB, small proportions of neuroblasts in the RMS that fail to reach their destination appear to undergo Bax-dependent PCD (Kim et al., 2007). Because ectopically located mature neurons in the RMS can impede neuroblasts migration in combination with glial tube defects, multiple factors may contribute to the impairment of RMS migration in Bax-KO mice. In NCAM-KO mice, defects in the RMS migration evoke massive PCD in the RMS via induction of p75-dependent apoptotic pathway (Gascon et al., 2007), supporting the idea that the migration-defective neuroblasts in the RMS are actively eliminated by Bax-dependent PCD. Following the olfactory nerve axotomy, increased PCD of SVZ-RMS cells were observed, thus raising a possibility that neural activity also modulate RMS migration, and neuroblasts that fail to normally migrate are ultimately removed by PCD (Mandairon et al., 2003). Active elimination of migration-defective neurons is also found during radial migration of Purkinje cells in normal or Reeler mice (Jung et al., 2008), suggesting that the removal of ectopically located neurons is a common utility of PCD during the brain development.
RMS is a specialized structure, and the regulation of neuroblast migration in the RMS is tightly regulated by various secretory and adhesive factors. Considering that SVZ is a major source of new cells in the adult brain, utilization of endogenous stem cells for brain regeneration may require the re-direction of migrating neuroblasts from their original destination to degenerated/damaged sites. Because affected neuronal populations are often selective in many neurodegenerative diseases, understanding the RMS migration and development of new strategy to utilize this will have significant impact on regenerative therapeutic approaches. Regeneration of the adult brain depends not only on a supply of appropriate numbers and types of neurons but also on the proper migration of neurons and reconstitution of the functionally suitable histoarchitecture. Therefore, much effort is required to understand the development of RMS and the regulation of RMS migration.
Figures and Tables
Fig. 1
Rostral migratory stream in the adult mouse brain. (A) New neurons born in the subventricular zone (SVZ) of the lateral ventricle (LV) migrate to the olfactory bulb (OB) through rostral migratory stream (RMS). The RMS tract is connected to sebependymal layer (SE), the central part of the OB. In the RMS, migrating the neuroblasts form chains and they are surrounded by glial tube. Within the RMS, parallel-running blood vessels provide additional scaffolds for migrating neuroblasts. (B, C) Double immunufluorescence labeling of migrating neuroblasts (red, DCX labeling) and glial tube (green, GFAP labeling) in the RMS. (B) shows parasagittal, and (C) shows coronal cut image. Abbreviations are: LV, lateral ventricle; CC, corpus callosum; Str, Stratum; A, type A neuroblasts; G, glial tube; V, blood vessels.
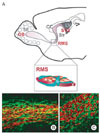
Fig. 2
Factors regulating RMS migration. In the SVZ, newly generated neuroblasts integrate into the cell clusters in chains and begin to migrate along the RMS (Step 1: Initiation). In the RMS, neuroblasts actively migrate to the rostral orientation, which is regulated by several factors including cell-cell/cell-ECM interactions, chemoattractive or chemorepulsive signals, and local environment in the RMS (Step 2: Migration). In the OB, migrated neuroblasts detach from chains, and they transfer to radial glial dependent-radial migration. By detachment from radial fiber, they arrive at their final destination (Step 3: Termination). Abbreviations are; ECM, extracellular matrix; SVZ, subventricular zone; RMS, rostral migratory stream; LV, lateral ventricle; CC, corpus callosum; Str, Stratum; SE, subependymal layer; A, type A neuroblasts; B, type B neural stem cells; C, type C transit-amplifying cells; E, ependymal cells; G, glial tube; V, blood vessels; R, radial fibers.
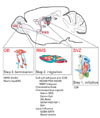
Acknowledgements
We dedicate this review to former Professor Nam, Sang Chae who tragically passed away by car accident at 2006. We thank Hyojin Yeo for reviewing our manuscript. This work was supported by grants from the Korean Ministry of Education, Science and Technology via Brain Research Center of the 21st century Frontier Program in Neuroscience (2010K000803) and National Research Foundation of Korea (20100020237).
References
1. Alonso M, Viollet C, Gabellec MM, Meas-Yedid V, Olivo-Marin JC, Lledo PM. Olfactory discrimination learning increases the survival of adult-born neurons in the olfactory bulb. J Neurosci. 2006. 26:10508–10513.
2. Andrade N, Komnenovic V, Blake SM, et al. ApoER2/VLDL receptor and Dab1 in the rostral migratory stream function in postnatal neuronal migration independently of Reelin. Proc Natl Acad Sci U S A. 2007. 104:8508–8513.
3. Angot E, Loulier K, Nguyen-Ba-Charvet KT, Gadeau AP, Ruat M, Traiffort E. Chemoattractive activity of sonic hedgehog in the adult subventricular zone modulates the number of neural precursors reaching the olfactory bulb. Stem Cells. 2008. 26:2311–2320.
4. Anton ES, Ghashghaei HT, Weber JL, et al. Receptor tyrosine kinase ErbB4 modulates neuroblast migration and placement in the adult forebrain. Nat Neurosci. 2004. 7:1319–1328.
5. Balenci L, Saoudi Y, Grunwald D, et al. IQGAP1 regulates adult neural progenitors in vivo and vascular endothelial growth factor-triggered neural progenitor migration in vitro. J Neurosci. 2007. 27:4716–4724.
6. Balordi F, Fishell G. Hedgehog signaling in the subventricular zone is required for both the maintenance of stem cells and the migration of newborn neurons. J Neurosci. 2007. 27:5936–5947.
7. Battista D, Rutishauser U. Removal of polysialic acid triggers dispersion of subventricularly derived neuroblasts into surrounding CNS tissues. J Neurosci. 2010. 30:3995–4003.
8. Bédard A, Parent A. Evidence of newly generated neurons in the human olfactory bulb. Brain Res Dev Brain Res. 2004. 151:159–168.
9. Belvindrah R, Hankel S, Walker J, Patton BL, Müller U. Beta1 integrins control the formation of cell chains in the adult rostral migratory stream. J Neurosci. 2007. 27:2704–2717.
10. Blake SM, Strasser V, Andrade N, et al. Thrombospondin-1 binds to ApoER2 and VLDL receptor and functions in postnatal neuronal migration. EMBO J. 2008. 27:3069–3080.
11. Bolteus AJ, Bordey A. GABA release and uptake regulate neuronal precursor migration in the postnatal subventricular zone. J Neurosci. 2004. 24:7623–7631.
12. Boutin C, Schmitz B, Cremer H, Diestel S. NCAM expression induces neurogenesis in vivo. Eur J Neurosci. 2009. 30:1209–1218.
13. Bovetti S, Bovolin P, Perroteau I, Puche AC. Subventricular zone-derived neuroblast migration to the olfactory bulb is modulated by matrix remodelling. Eur J Neurosci. 2007. 25:2021–2033.
14. Buss RR, Sun W, Oppenheim RW. Adaptive roles of programmed cell death during nervous system development. Annu Rev Neurosci. 2006. 29:1–35.
15. Cayre M, Bancila M, Virard I, Borges A, Durbec P. Migrating and myelinating potential of subventricular zone neural progenitor cells in white matter tracts of the adult rodent brain. Mol Cell Neurosci. 2006. 31:748–758.
16. Chazal G, Durbec P, Jankovski A, Rougon G, Cremer H. Consequences of neural cell adhesion molecule deficiency on cell migration in the rostral migratory stream of the mouse. J Neurosci. 2000. 20:1446–1457.
17. Chiaramello S, Dalmasso G, Bezin L, et al. BDNF/TrkB interaction regulates migration of SVZ precursor cells via PI3-K and MAP-K signalling pathways. Eur J Neurosci. 2007. 26:1780–1790.
18. Conover JC, Doetsch F, Garcia-Verdugo JM, Gale NW, Yancopoulos GD, Alvarez-Buylla A. Disruption of Eph/ephrin signaling affects migration and proliferation in the adult subventricular zone. Nat Neurosci. 2000. 3:1091–1097.
19. Coskun V, Luskin MB. Intrinsic and extrinsic regulation of the proliferation and differentiation of cells in the rodent rostral migratory stream. J Neurosci Res. 2002. 69:795–802.
20. Cremer H, Lange R, Christoph A, et al. Inactivation of the N-CAM gene in mice results in size reduction of the olfactory bulb and deficits in spatial learning. Nature. 1994. 367:455–459.
21. Curtis MA, Kam M, Nannmark U, et al. Human neuroblasts migrate to the olfactory bulb via a lateral ventricular extension. Science. 2007. 315:1243–1249.
22. Doetsch F. A niche for adult neural stem cells. Curr Opin Genet Dev. 2003. 13:543–550.
23. Emsley JG, Hagg T. alpha6beta1 integrin directs migration of neuronal precursors in adult mouse forebrain. Exp Neurol. 2003. 183:273–285.
24. Eom TY, Li J, Anton ES. Going tubular in the rostral migratory stream: neurons remodel astrocyte tubes to promote directional migration in the adult brain. Neuron. 2010. 67:173–175.
25. Garzotto D, Giacobini P, Crepaldi T, Fasolo A, De Marchis S. Hepatocyte growth factor regulates migration of olfactory interneuron precursors in the rostral migratory stream through Met-Grb2 coupling. J Neurosci. 2008. 28:5901–5909.
26. Gascon E, Vutskits L, Jenny B, Durbec P, Kiss JZ. PSA-NCAM in postnatally generated immature neurons of the olfactory bulb: a crucial role in regulating p75 expression and cell survival. Development. 2007. 134:1181–1190.
27. Giachino C, Taylor V. Lineage analysis of quiescent regenerative stem cells in the adult brain by genetic labelling reveals spatially restricted neurogenic niches in the olfactory bulb. Eur J Neurosci. 2009. 30:9–24.
28. Gotts JE, Chesselet MF. Migration and fate of newly born cells after focal cortical ischemia in adult rats. J Neurosci Res. 2005. 80:160–171.
29. Gritti A, Bonfanti L, Doetsch F, et al. Multipotent neural stem cells reside into the rostral extension and olfactory bulb of adult rodents. J Neurosci. 2002. 22:437–445.
30. Grubb MS, Nissant A, Murray K, Lledo PM. Functional maturation of the first synapse in olfaction: development and adult neurogenesis. J Neurosci. 2008. 28:2919–2932.
31. Hack I, Bancila M, Loulier K, Carroll P, Cremer H. Reelin is a detachment signal in tangential chain-migration during postnatal neurogenesis. Nat Neurosci. 2002. 5:939–945.
32. Hack MA, Saghatelyan A, de Chevigny A, et al. Neuronal fate determinants of adult olfactory bulb neurogenesis. Nat Neurosci. 2005. 8:865–872.
33. Hatten ME. Central nervous system neuronal migration. Annu Rev Neurosci. 1999. 22:511–539.
34. Hu H, Tomasiewicz H, Magnuson T, Rutishauser U. The role of polysialic acid in migration of olfactory bulb interneuron precursors in the subventricular zone. Neuron. 1996. 16:735–743.
35. Hurtado-Chong A, Yusta-Boyo MJ, Vergaño-Vera E, Bulfone A, de Pablo F, Vicario-Abejón C. IGF-I promotes neuronal migration and positioning in the olfactory bulb and the exit of neuroblasts from the subventricular zone. Eur J Neurosci. 2009. 30:742–755.
36. Jablonska B, Aguirre A, Raymond M, et al. Chordin-induced lineage plasticity of adult SVZ neuroblasts after demyelination. Nat Neurosci. 2010. 13:541–550.
37. Jankovski A, Garcia C, Soriano E, Sotelo C. Proliferation, migration and differentiation of neuronal progenitor cells in the adult mouse subventricular zone surgically separated from its olfactory bulb. Eur J Neurosci. 1998. 10:3853–3868.
38. Jung AR, Kim TW, Rhyu IJ, et al. Misplacement of Purkinje cells during postnatal development in Bax knock-out mice: a novel role for programmed cell death in the nervous system? J Neurosci. 2008. 28:2941–2948.
39. Kaneko N, Marín O, Koike M, et al. New neurons clear the path of astrocytic processes for their rapid migration in the adult brain. Neuron. 2010. 67:213–223.
40. Kessenbrock K, Plaks V, Werb Z. Matrix metalloproteinases: regulators of the tumor microenvironment. Cell. 2010. 141:52–67.
41. Kim HM, Qu T, Kriho V, et al. Reelin function in neural stem cell biology. Proc Natl Acad Sci U S A. 2002. 99:4020–4025.
42. Kim WR, Kim Y, Eun B, et al. Impaired migration in the rostral migratory stream but spared olfactory function after the elimination of programmed cell death in Bax knock-out mice. J Neurosci. 2007. 27:14392–14403.
43. Kirschenbaum B, Doetsch F, Lois C, Alvarez-Buylla A. Adult subventricular zone neuronal precursors continue to proliferate and migrate in the absence of the olfactory bulb. J Neurosci. 1999. 19:2171–2180.
44. Kohwi M, Osumi N, Rubenstein JL, Alvarez-Buylla A. Pax6 is required for making specific subpopulations of granule and periglomerular neurons in the olfactory bulb. J Neurosci. 2005. 25:6997–7003.
45. Kohwi M, Petryniak MA, Long JE, et al. A subpopulation of olfactory bulb GABAergic interneurons is derived from Emx1- and Dlx5/6-expressing progenitors. J Neurosci. 2007. 27:6878–6891.
46. Kornack DR, Rakic P. The generation, migration, and differentiation of olfactory neurons in the adult primate brain. Proc Natl Acad Sci U S A. 2001. 98:4752–4757.
47. Law AK, Pencea V, Buck CR, Luskin MB. Neurogenesis and neuronal migration in the neonatal rat forebrain anterior subventricular zone do not require GFAP-positive astrocytes. Dev Biol. 1999. 216:622–634.
48. Lee SR, Kim HY, Rogowska J, et al. Involvement of matrix metalloproteinase in neuroblast cell migration from the subventricular zone after stroke. J Neurosci. 2006. 26:3491–3495.
49. Li WL, Yu SP, Ogle ME, Ding XS, Wei L. Enhanced neurogenesis and cell migration following focal ischemia and peripheral stimulation in mice. Dev Neurobiol. 2008. 68:1474–1486.
50. Liu G, Rao Y. Neuronal migration from the forebrain to the olfactory bulb requires a new attractant persistent in the olfactory bulb. J Neurosci. 2003. 23:6651–6659.
51. Liu Z, Martin LJ. Olfactory bulb core is a rich source of neural progenitor and stem cells in adult rodent and human. J Comp Neurol. 2003. 459:368–391.
52. Lledo PM, Merkle FT, Alvarez-Buylla A. Origin and function of olfactory bulb interneuron diversity. Trends Neurosci. 2008. 31:392–400.
53. Lois C, Alvarez-Buylla A. Long-distance neuronal migration in the adult mammalian brain. Science. 1994. 264:1145–1148.
54. Lois C, García-Verdugo JM, Alvarez-Buylla A. Chain migration of neuronal precursors. Science. 1996. 271:978–981.
55. Mackay-Sima A, Chuahb MI. Neurotrophic factors in the primary olfactory pathway. Prog Neurobiol. 2000. 62:527–559.
56. Maina F, Klein R. Hepatocyte growth factor, a versatile signal for developing neurons. Nat Neurosci. 1999. 2:213–217.
57. Mandairon N, Jourdan F, Didier A. Deprivation of sensory inputs to the olfactory bulb up-regulates cell death and proliferation in the subventricular zone of adult mice. Neuroscience. 2003. 119:507–516.
58. Mandairon N, Sacquet J, Jourdan F, Didier A. Long-term fate and distribution of newborn cells in the adult mouse olfactory bulb: Influences of olfactory deprivation. Neuroscience. 2006. 141:443–451.
59. Marins M, Xavier AL, Viana NB, Fortes FS, Fróes MM, Menezes JR. Gap junctions are involved in cell migration in the early postnatal subventricular zone. Dev Neurobiol. 2009. 69:715–730.
60. Marshall CA, Novitch BG, Goldman JE. Olig2 directs astrocyte and oligodendrocyte formation in postnatal subventricular zone cells. J Neurosci. 2005. 25:7289–7298.
61. Mason HA, Ito S, Corfas G. Extracellular signals that regulate the tangential migration of olfactory bulb neuronal precursors: inducers, inhibitors, and repellents. J Neurosci. 2001. 21:7654–7663.
62. Merkle FT, Mirzadeh Z, Alvarez-Buylla A. Mosaic organization of neural stem cells in the adult brain. Science. 2007. 317:381–384.
63. Miragall F, Albiez P, Bartels H, de Vries U, Dermietzel R. Expression of the gap junction protein connexin43 in the subependymal layer and the rostral migratory stream of the mouse: evidence for an inverse correlation between intensity of connexin43 expression and cell proliferation activity. Cell Tissue Res. 1997. 287:243–253.
64. Mizrahi A. Dendritic development and plasticity of adult-born neurons in the mouse olfactory bulb. Nat Neurosci. 2007. 10:444–452.
65. Mouret A, Gheusi G, Gabellec MM, de Chaumont F, Olivo-Marin JC, Lledo PM. Learning and survival of newly generated neurons: when time matters. J Neurosci. 2008. 28:11511–11516.
66. Murase S, Cho C, White JM, Horwitz AF. ADAM2 promotes migration of neuroblasts in the rostral migratory stream to the olfactory bulb. Eur J Neurosci. 2008. 27:1585–1595.
67. Murase S, Horwitz AF. Deleted in colorectal carcinoma and differentially expressed integrins mediate the directional migration of neural precursors in the rostral migratory stream. J Neurosci. 2002. 22:3568–3579.
68. Nam SC, Kim Y, Dryanovski D, et al. Dynamic features of postnatal subventricular zone cell motility: a two-photon time-lapse study. J Comp Neurol. 2007. 505:190–208.
69. Nguyen-Ba-Charvet KT, Picard-Riera N, Tessier-Lavigne M, Baron-Van Evercooren A, Sotelo C, Chédotal A. Multiple roles for slits in the control of cell migration in the rostral migratory stream. J Neurosci. 2004. 24:1497–1506.
70. Olsson AK, Dimberg A, Kreuger J, Claesson-Welsh L. VEGF receptor signalling - in control of vascular function. Nat Rev Mol Cell Biol. 2006. 7:359–371.
71. Ono K, Takebayashi H, Ikenaka K. Olig2 transcription factor in the developing and injured forebrain; cell lineage and glial development. Mol Cells. 2009. 27:397–401.
72. Panzanelli P, Bardy C, Nissant A, et al. Early synapse formation in developing interneurons of the adult olfactory bulb. J Neurosci. 2009. 29:15039–15052.
73. Paratcha G, Ibáñez CF, Ledda F. GDNF is a chemoattractant factor for neuronal precursor cells in the rostral migratory stream. Mol Cell Neurosci. 2006. 31:505–514.
74. Pencea V, Bingaman KD, Freedman LJ, Luskin MB. Neurogenesis in the subventricular zone and rostral migratory stream of the neonatal and adult primate forebrain. Exp Neurol. 2001. 172:1–16.
75. Pencea V, Luskin MB. Prenatal development of the rodent rostral migratory stream. J Comp Neurol. 2003. 463:402–418.
76. Petreanu L, Alvarez-Buylla A. Maturation and death of adult-born olfactory bulb granule neurons: role of olfaction. J Neurosci. 2002. 22:6106–6113.
77. Platel JC, Dave KA, Bordey A. Control of neuroblast production and migration by converging GABA and glutamate signals in the postnatal forebrain. J Physiol. 2008. 586:3739–3743.
78. Powell EM, Mars WM, Levitt P. Hepatocyte growth factor/scatter factor is a motogen for interneurons migrating from the ventral to dorsal telencephalon. Neuron. 2001. 30:79–89.
79. Rice DS, Curran T. Role of the reelin signaling pathway in central nervous system development. Annu Rev Neurosci. 2001. 24:1005–1039.
80. Rochefort C, Gheusi G, Vincent JD, Lledo PM. Enriched odor exposure increases the number of newborn neurons in the adult olfactory bulb and improves odor memory. J Neurosci. 2002. 22:2679–2689.
81. Russo VC, Gluckman PD, Feldman EL, Werther GA. The insulin-like growth factor system and its pleiotropic functions in brain. Endocr Rev. 2005. 26:916–943.
82. Saghatelyan A, de Chevigny A, Schachner M, Lledo PM. Tenascin-R mediates activity-dependent recruitment of neuroblasts in the adult mouse forebrain. Nat Neurosci. 2004. 7:347–356.
83. Saghatelyan A, Roux P, Migliore M, et al. Activity-dependent adjustments of the inhibitory network in the olfactory bulb following early postnatal deprivation. Neuron. 2005. 46:103–116.
84. Saino-Saito S, Cave JW, Akiba Y. ER81 and CaMKIV identify anatomically and phenotypically defined subsets of mouse olfactory bulb interneurons. J Comp Neurol. 2007. 502:485–496.
85. Sawamoto K, Wichterle H, Gonzalez-Perez O, et al. New neurons follow the flow of cerebrospinal fluid in the adult brain. Science. 2006. 311:629–632.
86. Simó S, Pujadas L, Segura MF, et al. Reelin induces the detachment of postnatal subventricular zone cells and the expression of the Egr-1 through Erk1/2 activation. Cereb Cortex. 2007. 17:294–303.
87. Snapyan M, Lemasson M, Brill MS, et al. Vasculature guides migrating neuronal precursors in the adult mammalian forebrain via brain-derived neurotrophic factor signaling. J Neurosci. 2009. 29:4172–4188.
88. Soria JM, Taglialatela P, Gil-Perotin S, et al. Defective postnatal neurogenesis and disorganization of the rostral migratory stream in absence of the Vax1 homeobox gene. J Neurosci. 2004. 24:11171–11181.
89. Staquicini FI, Dias-Neto E, Li J, et al. Discovery of a functional protein complex of netrin-4, laminin gamma1 chain, and integrin alpha6beta1 in mouse neural stem cells. Proc Natl Acad Sci U S A. 2009. 106:2903–2908.
90. Sun W, Funakoshi H, Nakamura T. Localization and functional role of hepatocyte growth factor (HGF) and its receptor c-met in the rat developing cerebral cortex. Brain Res Mol Brain Res. 2002a. 103:36–48.
91. Sun W, Funakoshi H, Nakamura T. Overexpression of HGF retards disease progression and prolongs life span in a transgenic mouse model of ALS. J Neurosci. 2002b. 22:6537–6548.
92. Waclaw RR, Wang B, Pei Z, Ehrman LA, Campbell K. Distinct temporal requirements for the homeobox gene Gsx2 in specifying striatal and olfactory bulb neuronal fates. Neuron. 2009. 63:451–465.
93. Whitman MC, Fan W, Rela L, Rodriguez-Gil DJ, Greer CA. Blood vessels form a migratory scaffold in the rostral migratory stream. J Comp Neurol. 2009. 516:94–104.
94. Whitman MC, Greer CA. Adult neurogenesis and the olfactory system. Prog Neurobiol. 2009. 89:162–175.
95. Winner B, Cooper-Kuhn CM, Aigner R, Winkler J, Kuhn HG. Long-term survival and cell death of newly generated neurons in the adult rat olfactory bulb. Eur J Neurosci. 2002. 16:1681–1689.
96. Wittko IM, Schänzer A, Kuzmichev A, et al. VEGFR-1 regulates adult olfactory bulb neurogenesis and migration of neural progenitors in the rostral migratory stream in vivo. J Neurosci. 2009. 29:8704–8714.
97. Yamaguchi M, Mori K. Critical period for sensory experience-dependent survival of newly generated granule cells in the adult mouse olfactory bulb. Proc Natl Acad Sci U S A. 2005. 102:9697–9702.
98. Yamashita T, Ninomiya M, Hernández Acosta P, et al. Subventricular zone-derived neuroblasts migrate and differentiate into mature neurons in the post-stroke adult striatum. J Neurosci. 2006. 26:6627–6636.
99. Yang H, Jensen P, Goldowitz D. The community effect and Purkinje cell migration in the cerebellar cortex: analysis of scrambler chimeric mice. J Neurosci. 2002. 22:464–470.
100. Zhang SC. Defining glial cells during CNS development. Nat Rev Neurosci. 2001. 2:840–843.
101. Zigova T, Pencea V, Betarbet R, et al. Neuronal progenitor cells of the neonatal subventricular zone differentiate and disperse following transplantation into the adult rat striatum. Cell Transplant. 1998. 7:137–156.