Abstract
Reelin, an extracellular glycoprotein has an important role in the proper migration and positioning of neurons during brain development. Lack of reelin causes not only disorganized lamination of the cerebral and cerebellar cortex but also malpositioning of mesencephalic dopaminergic (mDA) neurons. However, the accurate role of reelin in the migration and positioning of mDA neurons is not fully elucidated. In this study, reelin-deficient reeler mice exhibited a significant loss of mDA neurons in the substantia nigra pars compacta (SNc) and a severe alteration of cell distribution in the retrorubal field (RRF). This abnormality was also found in Dab1-deficinet, yotari mice. Stereological analysis revealed that total number of mDA neurons was not changed compared to wild type, suggesting that the loss of mDA neurons in reeler may not be due to the neurogenesis of mDA neurons. We also found that formation of PSA-NCAM-positive tangential nerve fibers rather than radial glial fibers was greatly reduced in the early developmental stage (E14.5) of reeler. These findings provide direct evidence that the alteration in distribution pattern of mDA neurons in the reeler mesencephalon mainly results from the defect of the lateral migration using tangential fibers as a scaffold.
Reelin protein, encoded by the reelin gene (Reln), is an extracellular matrix glycoprotein that plays a critical role in the neuronal migration and positioning during development (for review see Rice & Curran, 2001). Reelin is secreted by specific neurons and binds to the very-low density lipoprotein receptor (VLDLR) and the ApoE receptor 2 (ApoER2) on other neurons (D'Arcangelo et al., 1999). A well-established signaling pathway of reelin involves binding to its receptors followed by tyrosine phosphorylation of the cytoplasmic adaptor Dab1, leading to cytoskeletal modifications and changes of gene expression in target neurons (Ballif et al., 2004). The reeler, caused by a disrupted reelin gene, manifests an abnormal laminar organization of several brain structures such as cerebral and cerebellar cortices and hippocampal formation (for review see, Förster et al., 2006). The loss of cellular organization in the cerebellum of reeler mice results in an ataxic phenotype characterized by tremors, dystonia, and a reeling gate. Another neurological mutant mouse, yotari, spontaneously arisen from a mutation in Dab1 gene also exhibits similar behavioral and anatomical traits with reeler mutant (Rice et al., 1998). Although most studies on reelin have focused on the regulatory functions in the migration and cell positioning in layered structures, recent studies also show that ectopic neurons are found in non-laminated structures, such as inferior olivary complex, trigeminal motor nucleus, substantia nigra, dorsal cochlear nucleus, and facial nucleus of reeler brain (Goffinet 1983; Terashima et al., 1994; Nishikawa et al., 2003; Takaoka et al., 2005; for review see, Katsuyama & Terashima, 2009).
Mesencephalic dopaminergic (mDA) neurons are distributed into three major cell groups: ventral tegmental area (VTA, A10), substantia nigra pars compacta (SNc, A9), and retrorubral field (RRF, A8) in the reticular formation (Hökfelt et al., 1984; German & Manaye, 1993). The mesencephalic A9 cells project to the dorsolateral striatum establishing the nigrostriatal pathway. The A10 and A8 cells innervate to the ventromedial striatum, amygdala, olfactory tubercle, thalamus, and hippocampus forming mesolimbic pathway (Björklund & Lindvall, 1984; Prakash & Wurst, 2006). Especially, the mDA output to the striatum plays a central role in motor and cognitive functions through distinct reciprocal fiber connections (Gerfen 1992). Recently, some studies provided evidence that embryonic striatal neurons may supply the ventral mesencephalon with reelin through their axonal projections (Nishikawa et al., 2003). During development, postmitotic DA neurons migrate away from the ventricular surface toward the ventromedial mesencephalon along radial glial fibers and then they migrate laterally along tangentially arranged nerve fibers (Kawano et al., 1995; Ohyama et al., 1998). However, our understanding on the correlation between malpositioning of mesencephalic DA neurons and the guiding fibers, such as radial glial fibers and tangential nerve fibers during development in reeler mice is still incomplete. Considering that a significant reduction in the process extension and the maturation of radial glial cells was observed during corticogenesis in reeler brain (Hunter-Schaedle 1997; Hartfuss et al., 2003), it is very attractive to investigate how the guiding fibers for the migration of mDA neurons are affected by reelin signal during development.
In the present study, we showed that the loss of mDA neurons in SNc and RRF in reeler mice may not be due to the neurogenesis of mDA neurons. Furthermore, we found that formation of tangential fibers rather than radial glial fibers was greatly suppressed in the reeler mesensephalon at the early developmental stage, indicating that alterations in distribution of mDA neurons in the reeler brain mainly result from the defect of interaction between migrating DA neurons and guiding fibers.
Homozygous reeler mice (Relnrl/rl) were produced by crossbreeding of heterozygous B6C3Fe-a/a-rl adults (Relnrl/+) purchased from the Jackson Laboratory (Bar Harbor ME, USA). Homozygous yotari mice (Dab1yot/yot) were bred from heteroozygous (Dab1yot/+) male and female mice which were kindly donated from Dr. N. Mitsuda (Ehime University, Japan). All mice were kept on a 14/10 hrs day/night cycle with food and water ad libitum. The morning on which a vaginal plug was detected was recorded as embryonic day 0.5 (E0.5) and day of birth as postnatal day 0 (P0). All experiments were carried out with the approval of the Committee on Animal Care and Welfare, Ajou University School of Medicine.
The genetic background of the reeler mice was confirmed by detection of the reelin breakpoint (D'Arcangelo et al., 1996). All primers were synthesized by Bioneer (Seoul, Korea). Modified primer sequences for reeler were as follows; Fwt/rl (forward), 5'-TTA ATC TGT CCT CAC TCT GCC CTC T-3'; Rwt (reverse), 5'-ATA AAA ACA GGA ATG AAG CAG ACT C-3'; Rrl (reverse), 5'-TTC CTC TCT TGC ATC CTG TTT TG-3'. For genotyping of yotari mice, following primers were used (Kojima et al., 2000); Fwt/dab1 (forward), 5'-GCC CTT CAG CAT CAC CAT GCT-3'; Rdab1 (reverse), 5'-CAG TGA GTA CAT ATT GTG TGA GTT CC-3'; Rwt (reverse), 5'-CCT TGT TTC TTT GCT TTA AGG CTG T-3'.
Pups of stage E14.5, E16.5, P0, P3, and P7 were used in this study. In the case of embryo, the whole heads were removed in ice-cold calcium-magnesium-free buffered saline, fixed overnight in 4% paraformaldehyde in 0.1 M phosphate buffer (pH 7.4), and then were kept overnight in 30% sucrose at 4℃ for cryoprotection. They were frozen in dry ice powder and then 30 µm coronal sections were cut with freezing sliding microtome (Leica, Nussloch, Germany). Postnatal mice were deeply anesthetized with a combination of ketamin (50 mg/kg) and lumpun (2.5 mg/kg) and transcardially perfused with 0.9% saline in 0.1 M phosphate buffer (PBS, pH 7.4) followed by 4% paraformaldehyde in 0.1 M phosphate buffer (pH 7.4). The brains were removed and post-fixed with the same fixative at 4℃ overnight, cryoprotected in 30% sucrose at 4℃ overnight, and then frozen in dry ice. For stereological analysis, series of 50 µm coronal sections were cut.
The sections were blocked in 10% normal goat or rabbit serum containing 0.1% BSA in PBS for 30 min. To label mDA neurons, sections were incubated in polyclonal rabbit anti-TH (tyrosine hydroxylase) (Pel-Freez Biologicals, Rogers, AR, USA) diluted 1 : 1,000 in blocking solution for 24 hr at 4℃. Monoclonal mouse anti-RC2 (Hybridoma Bank, Iowa, IA, USA) diluted 1 : 50 and monoclonal mouse anti-BLBP (brain lipid binding protein) (Abcam, Cambridge, UK) diluted in 1 : 1,000 were used to visualize the radial glial fibers. To label the tangential nerve fibers in the developing mesencephalon, monoclonal mouse anti-PSA-NCAM (polysialylated neuronal cell adhesion molecule) (Chemicon/Millipore, Temecula, CA, USA) diluted in 1 : 500 was used. After incubation with primary antibody, sections were subsequently incubated with Alexa Fluor 488-conjugated anti-mouse or anti-rabbit secondary antibodies (Molecular Probes, Eugene, OR, USA) diluted in 1 : 200 and counterstained with bis-benzamide (Molecular Probes). Fluorescent images were acquired using a Carl Zeiss LSM710 confocal microscope (Zeiss, Jena, Germany). Alternatively, the sections were incubated with the biotin-conjugated secondary antibody diluted in 1 : 500 and then avidin-biotin complex using ABC kit (Vector Laboratories, Burlingame, CA, USA) following the manufacturer's protocol. Finally, immunoreaction was visualized with 0.05% 3,3'-diaminobezidine and 0.03% hydrogen peroxide substrate solution. The brightfield images were acquired using an Axiophot microscope (Zeiss).
To determine the total number of midbrain DA neurons, 50 µm thick frozen sections were obtained from P7 mice and immunostained with rabbit anti-TH antibody as described above. Optical fractionator sampling was carried out in an Olympus BX51 microscope (Olympus, Tokyo, Japan) equipped with a motorized stage (MicroBrightField, Colchester, VT, USA). Sampling was implemented using the Stereoinvestigator software package (MicroBrightField). According to the mouse brain atlas of Paxinos & Franklin, (2001), the substantia nigra (SNc), ventral tegmental area (VTA), and retrorubral field (RRF) regions were outlined. In order for our counting to encompass the full rostrocaudal extent of the relevant midbrain DA neurons, 12 sections from 1 : 3 series were analyzed for each brain. Prior to beginning counting a section, average thickness was measured. A guard height of 4 µm was used with a sampling brick depth of 20 µm. Counting frame information: counting frame width (X) was 100 µm, height (Y) was 100 µm, and depth was 20 µm. Guard regions of 4 µm thick were used for counting frame depth. Counting frame was maintained across all regions sampled. Sampling grid size was equal for major regions (VTA, SNc, and RRF). The sizes were: width (X) 253 µm, height (Y) 135 µm. A cell was only marked and counted if a nucleus surrounded by cytoplasm filled with a colored precipitate was clearly visible. Cells were counted only if they came into focus when racking the focus down through the sampling brick.
Midbrain tyrosine hydroxylase (TH)-positive DA neurons are located in three cell groups; ventral tegmental area (VTA, A10), substantia nigra pars compacta (SNc, A9), and retrorubral field (RRF, A8) (German & Manaye, 1993). To demonstrate the altered distribution of DA neurons in the reeler mesencephalon, we performed immunostaining for TH using serial coronal sections of whole midbrains of P0 and P7. In the reeler mensencephalon, the most striking morphological alteration was a significant loss of mDA neurons in the substantia nigra pars compacta (SNc) and substantia nigra lateralis (SNL) (arrow in Fig. 1Ab). The retrorubral field of reeler, in contrast to wild type, showed a widely diffused distribution of mDA neurons without forming a discrete cell group (asterisk in Fig. 1Ae). These abnormal distribution patterns in reeler mice was very similar with yotari mice lacking dab1 adaptor protein associated with reelin receptors (arrow in Fig. 1Ac and asterisk in Fig. 1Af), implying that this histological alterations are due to the defect of reelin-dab1 signaling. Interestingly, the ectopic clusters of mDA neurons were seen in the lateral border of the VTA, the region of parabrachial nuclei in reeler and yotari (arrows in Fig. 1Be, f). Considering that mDA neurons in the SNc and RRF come from the VTA by the lateral migration (Ohyama et al., 1998), this finding gave an impression that disturbance of lateral migration may be concerned in the morphological abnormality of the reeler mesencephalon.
As explanation for the remarked reduction of mDA neurons in the SNc of reeler, we postulated two possibilities; one is the defect of generation and differentiation of DA neurons destined to the mesencephalon during early development and the other is the incomplete neuronal migration to the final position. To address this question, we estimated the number of TH-positive DA neurons in the VTA, SNc, and RRF. Although the VTA is known to be composed of the caudal linear nucleus of raphe, interfascicular, parainterfascicular, parabrachial, paranigral, and rostral linear subnuclei (Paxinos & Franklin, 2001; Nair-Roberts et al., 2008), we counted the cells in the entire VTA without further subdivision of nuclei (Fig. 2A). As shown in Fig. 2B, there was marked decrease in the number of TH-positive neurons in both SNc (wild type: 2,058±383, reeler: 724±55) and RRF (wild type: 1,166±106, reeler: 788±194) of reeler mice. In contrast, the number of TH-positive neurons in the VTA in reeler (7,264±57) was significantly increased compared with wild type (5,852±21). Noteworthy was that total number of TH-positive neurons in these three major cell groups in reeler (8,776±265) was almost same as that in wild type (9,076±346). That is, the proportion of TH-positive neurons in the VTA of reeler was much higher than in wild type, but those in the SNc and RRF was much lower (Fig. 2C). This result increases the possibility that the main cause of abnormality in reeler may be a failure of the migration of mDA neurons destined for SNc and RRF rather than a defect of neurogenesis in the ventricular zone.
To demonstrate whether the migratory defect of mDA neurons is correlated with guidance fibers, we firstly examined formation of radial glial fibers using immunostaining for radial glial markers, RC2 and BLBP. The mDA neurons begin to segregate into the SNc and the VTA around E14-E15 in the mouse (Hu et al., 2004). Therefore, we examined the radial glial system in the mesencephalon at E14.5 and E16.5. At E14.5, RC2-positive radial glial fibers extend from the ventricular surface to the pial surface of the mesencephalon. Notably, there was no difference between wild type and reeler mice (Fig. 3A, B). In contrast, the development of RC2-positive fibers in the reeler mesencephalon at E16.5 was prominently reduced (Fig. 3C, D). The distribution pattern of radial fibers in RC2 immunohistochemistry coincided with the BLBP immunostaining result (Fig. 4). The depressed arrangement of BLBP-positive ralial glial fibers in reeler was also found at the stage of E16.5, not E14.5. These results indicate that the radial migration from the ventricular surface to the ventral mesencephalon, which occurred prior to the segregation of nigral DA neurons from the VTA, may not be affected by reelin.
Mesencephalic DA neurons migrate to the SNc, RRF, and VTA in two phases; the radial migration and subsequent lateral migration using radial glial fibers and tangential nerve fibers as guiding scaffold, respectively. We examined the reelin effect on tangential nerve fibers using immunostaining for PSA-NCAM, which is continuously expressed in tangentially arranged nerve fibers during the lateral migratory period (Ohyama et al., 1998). While abundant PSA-NCAM-positive fibers are horizontally arranged in the RRF, caudal region of the mesencephalon, in E14.5 wild type, a significant reduction of tangential fibers was seen in reeler mice (Fig. 5). Taken together with the result on radial glial fibers at the same stage, poor organization of the scaffold system essential for the lateral migration may be a main cause of the morphological defect in reeler mesencephalon.
In dab1-deficient yotari mice, we found altered distribution of mDA neurons being almost identical with reeler. When compared guiding fibers at E14.5 in reeler and yotari, as seen in Fig. 6, developmental pattern of RC2- and BLBP-positive radial glial fibers as well as PSA-NCAM-positive fibers in yotari was very similar with those in reeler mice.
During embryonic development, mesencephalic dopaminergic (mDA) neurons which are generated in the ventricular zone migrate to form the ventral tegmental area (VTA; A10), substantia nigra pars compacta (SNc; A9), and retrorubral field (RRF; A8). The migratory mode of mDA neurons is divided into two phases (Shults et al., 1990; Kawano et al., 1995; Ohyama et al., 1998). First, the young mDA neurons from the ventricular surface migrate to the ventromedial mesencephalon along radial glial fibers. Second, they migrate laterally in the basal part of ventral mesencephalon along tangentially arranged nerve fibers to form the anatomically specified cell groups. In the present study, we demonstrated a great loss of mDA neurons in the SNc and widely diffused distribution of RRF neurons in reeler mice. Our observation was consistent with previous report (Nishikawa et al., 2003). Another neurological mutant, dab1-deficient yotari showed almost same histological phenotype with reeler, confirming that malpositioning of mDA neurons occurs by the absence of reelin-dab1 siganaling. The loss of mDA neurons in the SNc of reeler is not thought to be related with the defect of generation of mDA neurons destined for the SNc, because of our finding showing the total number of mDA neurons in reeler was almost same as normal. Furthermore, a significant increase in VTA neurons and the ectopic cell cluster found in the lateral border of the VTA in reeler can be interpreted as a result of the defect of the lateral migration in the ventral mesencephalon.
The issue of whether reelin-dab1 signaling is involved in the cell fate decision during brain development remains to be clarified. Recently some studies displayed a decrease in the number of newly generated neurons and an increase in astrocytes in adult reeler (Won et al., 2006; Zhao et al., 2007) and developing dab1-deficient mice (Kwon et al., 2009). Based on these observations, our result showing the total number of mDA neurons in the absence of reelin was not different from that of wild type is unexpected. However, we cannot exclude the possibility that reelin effect on neurogenesis may be somewhat different depending on the neuronal cell type.
During brain development, reelin is highly expressed in the ventral mesenceohalon at E15, when nigral DA neurons proceed to migrate laterally to their final positions (Nishikawa et al., 2003). At this stage, reelin is known to be supplied by striatal patch neurons via axonal transport system (Nishikawa et al., 1999). Up to now, precise function of reelin has been a controversial issue in brain development. Many investigators have proposed that reelin might act as a stop signal for migrating neurons (Curran & D'Arcangelo, 1998; Dulabon et al., 2000; for review see Rice & Curran, 2001). However, some studies suggested favorable evidences for an attractant function of reelin (Gilmore & Herrup, 2000). Considering our result showing that the accumulation of mDA neurons in the ventral mesencephalon at E14.5 was not impaired despite of the absence of reelin, it is more plausible that reelin might play a role as a stop signal in the developing mesencephalon. On the other hand, altered distribution of mDA neurons in reeler may be explained by the hypothesis that reelin acts as a detachment signal, not a stop or guidance cue (Hack et al., 2002; Magdaleno et al., 2002). According to this argument, we can postulate that reeler mDA neurons in the ventral mesencephalon cannot be easily separated from radial glial process, resulting in the failure of subsequent lateral migration to their destination sites.
Although we did not perform the axon tracing, it would be interesting to see whether axonal projections from mDA neurons are changed by neuronal malpositioning in reeler mice. During development of hippocampus and spinal cord, misplaced neurons in reeler mutant exhibit specificity of synaptic connections is maintained (Deller et al., 1999; Yip et al., 2003). In the mesencephalon, axonal projections from nigral DA neurons preferentially innervate striatal patches forming the dopamine islands composed of axon terminals from nigral DA neurons. In reeler mice, the formation of striatal dopamine islands is almost normal, suggesting that nigral DA neurons can project axons to the striatum, despite their misplacement (Nishikawa et al., 2003).
As well as the regional concentration of reelin, its effect on the guiding fiber system is another important issue to understand the neuronal migration. The antibody against RC2, a member of intermediate filament associated protein (IFAP), has been frequently used to label radial glial cells both in vivo and in vitro (Noctor et al., 2002; Pollard & Conti, 2007). Brain lipid binding protein (BLBP) is a member of the large family of hydrophobic ligand binding proteins and expressed in radial glia and astrocyte during CNS development (Feng et al., 1994; Hartfuss et al., 2001). Many previous studies showed a significant reduction in the process extension and maturation of radial glial cells during corticogenesis in reeler mutant (Hartfuss et al., 2003; Keilani & Sugaya, 2008). Interestingly, we could not observe any difference in radial glial fibers development between reeler and wild type at E14.5, although a prominent decrease was seen at E16.5. In mouse development, TH-expressing cells are first appeared in the neuroepithelium at E10 and they are located laterally along the ventral pial surface by E14 (Kawano et al., 1995; Martín et al., 2005). Subsequently, mDA neurons in the ventral mesencephalon begin to separate into the SN and the VTA around E14-E15 (Hu et al., 2004). Based on these observations, the radial migration of mDA neurons in the developing mesencephalon is considered to proceed actively, at least, before E15. Thus, our RC2 and BLBP immunostaining results on E14.5 suggest that the radial migration may not be markedly affected by reelin before E15. This speculation is supported by our observation that total number of mDA neurons in the VTA was not decreased. It has been known that radial glial cells are transformed into astrocytes at the end of the neuronal migration (Hunter-Schaedle 1997). Furthermore, the absence of reelin accelerates the transformation of radial glial cells into astrocytes. (Super et al., 2000; Weiss et al., 2003). From this view, the regressive change of radial glial fibers in E16.5 reeler might be related to the early transformation of radial glia to astrocytes.
PSA-NCAM was expressed on tangential nerve fibers in the ventral mesencephalon throughout E11-E13 (Ohyama et al., 1998). The poor arrangement of tangential fibers was seen in both E14.5 reeler and yotari mice, suggests that malpositioning of mDA neurons in both mutants is mainly due to the defect of tangential nerve fibers rather than radial glial fibers. In corticogenesis, tangential migration of a subpopulation of projection neurons is disrupted in reeler mice, but tangential migration of inhibitory interneurons originated in the ganglionic eminence is not influenced by reelin mutation (Britanova et al., 2006). Little is known about the molecular mechanism that regulates the differentiation or guidance roles of tangential nerve fibers. Recent evidence indicates that a deficiency of p35, a regulator of Cdk5 involved in reelin signaling (Ohkubo et al., 2002), induces retarded tangential migration and delayed cortical entry of interneurons (Rakić et al., 2009). Our previous study using neural stem cells showed that Dab1 deficiency suppressed the expression of NeuroD, a transcription factor for neuronal differentiation, but significantly increased the GFAP expression (Kwon et al., 2009). Therefore we expect the possibility that reelin-dab1 signaling is involved in the regulation of tangential nerve fiber differentiation. However, underlying molecular mechanism of reelin effects on the differentiation of neurons acting as tangential scaffolds and on their axon growth awaits a further investigation. In the present study, we have presented three findings in developing reeler mesencephalon: first, the loss of mDA neurons in the SNc and RRF is not related with the neurogenesis, second, the early radial migration is not influenced by reelin, and finally, malformation of tangential nerve fibers is responsible for abnormal positioning of mDA neurons.
Figures and Tables
Fig. 1
Abnormal positioning of mDA neurons in reeler and yotari mice at P0. (A) DA cells labeled with TH-immunoractivity are significantly reduced in the substantia nigra pars compacta (SNc) and lateralis (SNL) of reeler and yotari mouse (arrows in b and c). In retrorubral field (RRF), unlike to wild type, mDA neurons are diffusely distributed in the region between the vental tegmental area (VTA) and the RRF of reeler and yotari mice (asterisks in e and f). (B) Characteristically, the ectopic cluster of mDA neurons in the lateral border of the VTA is observed in reeler and yotari (arrows in e and f). Scale bar=50 µm (a, d in A, d in B), 500 µm (a in B).
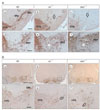
Fig. 2
Stereologic analysis of the numbers of mDA neurons in the VTA, SNc, and RRF at P7. (A) Borders of the VTA, SNc, and RRF were designated based on the mouse brain atlas of Paxinos & Franklin (2001). To encompass the full rostrocaudal extent of the midbrain DA neurons, 12 sections from 1 : 3 series were analyzed for each brain. (B) In reeler mice, the number of DA neurons is significantly reduced in the SNC and RRF, but rather VTA cells are increased. It is noticed that the total number of mDA neurons in these major cell groups of reeler is almost same as that in wild type. (C) The pie charts show the decrease in the percentage of mDA neurons in the SNc and RRF of reeler. VTA, vental tegmental area; SNc, substantia nigra pars compacta; RRF, retrorubral field. Data were obtained from three experiments; values are means±SEM. 12 sections from a 1 in 3 series were analyzed per brain. Statistical analysis was performed using t-test compared with wild type. n.s, not significant.
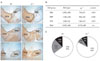
Fig. 3
Immunofluorescence staining for TH (red) and RC2 (green) in the ventral mesencephalon at E14.5 (A, B) and E16.5 (C, D). In the ventral mesencephalon at E14.5, RC2-positive cells and fibers in upper (a) and lower (b) regions of the midline and the lateral region (c) do not show a distinct difference between wild type and reeler. However, at E16.5, RC2 immunoreactivity is markedly reduced in reeler compared to E14.5. Especially, a poor formation of RC2-positive radial glial fibers extending to the pial surface is noticed in reeler mutant (c in D). The lack of distribution of mDA neurons in the lateral region of the ventral mesencephalon at E16.5 is noted (arrows). Scale bar=500 µm (A) and 50 µm (a, b, c in A).
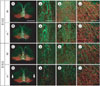
Fig. 4
Immunofluorescence staining for BLBP (green) in the ventral mesencephalon of E14.5 (A, B) and E16.5 (C, D). At E14.5, BLBP-positive radial fibers in reeler do not show a difference compared to wild type. However, at E16.5, the number of BLBP-positive radial glial fibers in reeler is greatly reduced, parallel to RC2-positive radial glial fibers (c of D in Fig. 3). Scale bar=500 µm (A, low magnification image) and 50 µm (enlarged image of A).
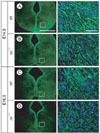
Fig. 5
Immunofluorescence staining for TH (red) and PSA-NCAM (green) in the RRF, caudal regions of the mesencephalon at E14.5. This figure shows different two levels of the RRF (A, C and B, D). Although, formation of RC2- or BLBP-positive radial fibers in reeler at the same stage was not different from wild type (as shown in Fig. 3 and Fig. 4), PSA-NCAM-positive tangential nerve fibers in the RRF are weakly organized compared to wild type. Scale bar: 500 µm (A, low magnification image) and 50 µm (enlarged image of A).
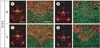
Fig. 6
Immunostaining of radial glial fibers and tangential fibers in E16.5 reeler and yotari mice. The reduction of radial glial fibers formation in reeler is also seen in yotari mice (B, C and E, F). Furthermore, poor development of PSA-NCAM-positive tangential nerve fibers is noticed in both reeler and yotari mutants (arrows in H, I). Scale bar=50 µm (A, D, G).
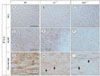
Acknowledgements
This work was supported by the "GRRC" project of Gyeonggi Provincial Government, Republic of Korea.
References
1. Ballif BA, Arnaud L, Arthur WT, Guris D, Imamoto A, Cooper JA. Activation of a Dab1/CrkL/C3G/Rap1 pathway in reelin-stimulated neurons. Curr Biol. 2004. 14:606–610.
2. Björklund A, Lindvall O. Björklund A, Hökfelt T, editors. Dopamine-containing systems in the CNS. Handbook of Chemical Neuroanatomy. 1984. Vol. 2. Amsterdam: Elsevier;55–121.
3. Britanova O, Alifragis P, Junek S, Jones K, Gruss P, Tarabykin V. A novel mode of tangential migration of cortical projection neurons. Dev Biol. 2006. 298:299–311.
4. Curran T, D'Arcangelo G. Role of reelin in the control of brain development. Brain Res Brain Res Rev. 1998. 26:285–294.
5. D'Arcangelo G, Homayouni R, Keshvara L, Rice DS, Sheldon M, Curran T. Reelin is a ligand for lipoprotein receptors. Neuron. 1999. 24:471–479.
6. D'Arcangelo G, Miao GG, Curran T. Detection of the reelin breakpoint in reeler mice. Brain Res Mol Brain Res. 1996. 39:234–236.
7. Deller T, Drakew A, Frotscher M. Different primary target cells are important for fiber lamination in the fascia dentata: a lesson from reeler mutant mice. Exp Neurol. 1999. 156:239–253.
8. Dulabon L, Olson EC, Taglienti MG, et al. Reelin binds alpha3beta1 integrin and inhibits neuronal migration. Neuron. 2000. 27:33–44.
9. Feng L, Hatten ME, Heintz N. Brain lipid-binding protein (BLBP): a novel signaling system in the developing mammalian CNS. Neuron. 1994. 12:895–908.
10. Förster E, Jossin Y, Zhao S, Chai X, Frotscher M, Goffinet AM. Recent progress in understanding the role of Reelin in radial neuronal migration, with specific emphasis on the dentate gyrus. Eur J Neurosci. 2006. 23:901–909.
11. Gerfen CR. The neostriatal mosaic: multiple levels of compartmental organization in the basal ganglia. Annu Rev Neurosci. 1992. 15:285–320.
12. German DC, Manaye KF. Midbrain dopaminergic neurons (nuclei A8, A9, and A10): three-dimensional reconstruction in the rat. J Comp Neurol. 1993. 331:297–309.
13. Gilmore EC, Herrup K. Cortical development: receiving reelin. Curr Biol. 2000. 10:R162–R166.
14. Goffinet AM. The embryonic development of the inferior olivary complex in normal and reeler (rlORL) mutant mice. J Comp Neurol. 1983. 219:10–24.
15. Hack I, Bancila M, Loulier K, Carroll P, Cremer H. Reelin is a detachment signal in tangential chain-migration during postnatal neurogenesis. Nat Neurosci. 2002. 5:939–945.
16. Hartfuss E, Förster E, Bock HH, et al. Reelin signaling directly affects radial glia morphology and biochemical maturation. Development. 2003. 130:4597–4609.
17. Hartfuss E, Galli R, Heins N, Götz M. Characterization of CNS precursor subtypes and radial glia. Dev Biol. 2001. 229:15–30.
18. Hökfelt T, Martensson R, Björklund A, Kleinau S, Goldstein M. Björklund A, Hökfelt T, editors. Distributional maps of tyrosine-hydroxylase-immunoreactive neurons in the rat brain. Handbook of Chemical Neuroanatomy. 1984. Vol. 2. Amsterdam: Elsevier;277–379.
19. Hu Z, Cooper M, Crockett DP, Zhou R. Differentiation of the midbrain dopaminergic pathways during mouse development. J Comp Neurol. 2004. 476:301–311.
20. Hunter-Schaedle KE. Radial glial cell development and transformation are disturbed in reeler forebrain. J Neurobiol. 1997. 33:459–472.
21. Katsuyama Y, Terashima T. Developmental anatomy of reeler mutant mouse. Dev Growth Differ. 2009. 51:271–286.
22. Kawano H, Ohyama K, Kawamura K, Nagatsu I. Migration of dopaminergic neurons in the embryonic mesencephalon of mice. Brain Res Dev Brain Res. 1995. 86:101–113.
23. Keilani S, Sugaya K. Reelin induces a radial glial phenotype in human neural progenitor cells by activation of Notch-1. BMC Dev Biol. 2008. 8:69.
24. Kojima T, Nakajima K, Mikoshiba K. The disabled 1 gene is disrupted by a replacement with L1 fragment in yotari mice. Brain Res Mol Brain Res. 2000. 75:121–127.
25. Kwon IS, Cho SK, Kim MJ, et al. Expression of disabled 1 suppresses astroglial differentiation in neural stem cells. Mol Cell Neurosci. 2009. 40:50–61.
26. Magdaleno S, Keshvara L, Curran T. Rescue of ataxia and preplate splitting by ectopic expression of Reelin in reeler mice. Neuron. 2002. 33:573–586.
27. Marín F, Herrero MT, Vyas S, Puelles L. Ontogeny of tyrosine hydroxylase mRNA expression in mid- and forebrain: neuromeric pattern and novel positive regions. Dev Dyn. 2005. 234:709–717.
28. Nair-Roberts RG, Chatelain-Badie SD, Benson E, White-Cooper H, Bolam JP, Ungless MA. Stereological estimates of dopaminergic, GABAergic and glutamatergic neurons in the ventral tegmental area, substantia nigra and retrorubral field in the rat. Neuroscience. 2008. 152:1024–1031.
29. Nishikawa S, Goto S, Hamasaki T, Ogawa M, Ushio Y. Transient and compartmental expression of the reeler gene product reelin in the developing rat striatum. Brain Res. 1999. 850:244–248.
30. Nishikawa S, Goto S, Yamada K, Hamasaki T, Ushio Y. Lack of Reelin causes malpositioning of nigral dopaminergic neurons: evidence from comparison of normal and Reln(rl) mutant mice. J Comp Neurol. 2003. 461:166–173.
31. Noctor SC, Flint AC, Weissman TA, Wong WS, Clinton BK, Kriegstein AR. Dividing precursor cells of the embryonic cortical ventricular zone have morphological and molecular characteristics of radial glia. J Neurosci. 2002. 22:3161–3173.
32. Ohkubo N, Lee YD, Morishima A, et al. Apolipoprotein E and Reelin ligands modulate tau phosphorylation through an apolipoprotein E receptor/disabled-1/glycogen synthase kinase-3beta cascade. FASEB J. 2002. 17:295–297.
33. Ohyama K, Kawano H, Asou H, et al. Coordinate expression of L1 and 6B4 proteoglycan/phosphacan is correlated with the migration of mesencephalic dopaminergic neurons in mice. Brain Res Dev Brain Res. 1998. 107:219–226.
34. Paxinos G, Franklin KBJ. The mouse brain in stereotaxic coordinates. 2001. 2nd ed. San Diego: Academic Press.
35. Prakash N, Wurst W. Development of dopaminergic neurons in the mammalian brain. Cell Mol Life Sci. 2006. 63:187–206.
36. Pollard SM, Conti L. Investigating radial glia in vitro. Prog Neurobiol. 2007. 83:53–67.
37. Rakić S, Yanagawa Y, Obata K, Faux C, Parnavelas JG, Nikolić M. Cortical interneurons require p35/Cdk5 for their migration and laminar organization. Cereb Cortex. 2009. 19:1857–1869.
38. Rice DS, Curran T. Role of the reelin signaling pathway in central nervous system development. Annu Rev Neurosci. 2001. 24:1005–1039.
39. Rice DS, Sheldon M, D'Arcangelo G, Nakajima K, Goldowitz D, Curran T. Disabled-1 acts downstream of Reelin in a signaling pathway that controls laminar organization in the mammalian brain. Development. 1998. 125:3719–3729.
40. Shults CW, Hashimoto R, Brady RM, Gage FH. Dopaminergic cells align along radial glia in the developing mesencephalon of the rat. Neuroscience. 1990. 38:427–436.
41. Super H, Del Rio JA, Martinez A, Perez-Sust P, Soriano E. Disruption of neuronal migration and radial glia in the developing cerebral cortex following ablation of Cajal-Retzius cells. Cereb Cortex. 2000. 10:602–613.
42. Takaoka Y, Setsu T, Misaki K, Yamauchi T, Terashima T. Expression of reelin in the dorsal cochlear nucleus of the mouse. Brain Res Dev Brain Res. 2005. 159:127–134.
43. Terashima T, Kishimoto Y, Ochiishi T. Musculotopic organization in the motor trigeminal nucleus of the reeler mutant mouse. Brain Res. 1994. 666:31–42.
44. Weiss KH, Johanssen C, Tielsch A, et al. Malformation of the radial glial scaffold in the dentate gyrus of reeler mice, scrambler mice, and ApoER2/VLDLR-deficient mice. J Comp Neurol. 2003. 460:56–65.
45. Won SJ, Kim SH, Xie L, et al. Reelin-deficient mice show impaired neurogenesis and increased stroke size. Exp Neurol. 2006. 198:250–259.
46. Yip YP, Rinaman L, Capriotti C, Yip JW. Ectopic sympathetic preganglionic neurons maintain proper connectivity in the reeler mutant mouse. Neuroscience. 2003. 118:439–450.
47. Zhao S, Chai X, Frotscher M. Balance between neurogenesis and gliogenesis in the adult hippocampus: role for reelin. Dev Neurosci. 2007. 29:84–90.