Abstract
Purpose
Several morphometric studies have been performed to investigate brain abnormalities in congenitally deaf people. But no report exists concerning structural brain abnormalities in congenitally deaf adolescents. We evaluated the regional volume changes in gray matter (GM) using voxel-based morphometry (VBM) in congenitally deaf adolescents.
Materials and Methods
A VBM8 methodology was applied to the T1-weighted magnetic resonance imaging (MRI) scans of eight congenitally deaf adolescents (mean age, 15.6 years) and nine adolescents with normal hearing. All MRI scans were normalized to a template and then segmented, modulated, and smoothed. Smoothed GM data were tested statistically using analysis of covariance (controlled for age, gender, and intracranial cavity volume).
Results
The mean values of age, gender, total volumes of GM, and total intracranial volume did not differ between the two groups. In the auditory centers, the left anterior Heschl's gyrus and both inferior colliculi showed decreased regional GM volume in the congenitally deaf adolescents. The GM volumes of the lingual gyri, nuclei accumbens, and left posterior thalamic reticular nucleus in the midbrain were also decreased.
Conclusions
The results of the present study suggest that early deprivation of auditory stimulation in congenitally deaf adolescents might have caused significant underdevelopment of the auditory cortex (left Heschl's gyrus), subcortical auditory structures (inferior colliculi), auditory gain controllers (nucleus accumbens and thalamic reticular nucleus), and multisensory integration areas (inferior colliculi and lingual gyri). These defects might be related to the absence of general auditory perception, the auditory gating system of thalamocortical transmission, and failure in the maturation of the auditory-to-limbic connection and the auditorysomatosensory-visual interconnection.
To investigate brain abnormalities in congenitally deaf people, several morphometric studies have been performed (1, 2, 3, 4, 5). However, no report exists concerning structural brain abnormalities in congenitally deaf adolescents (aged 13-17 years). Additionally only a few studies have revealed structural abnormalities in the auditory cortex (1, 4) and cochlear nerve (6) whereas none has reported structural deficits in the subcortical auditory centers. Those previous voxel-based morphometry (VBM) studies in deaf adults have generally reported no significant change in gray matter (GM) despite focal white matter (WM) deficits in the left posterior superior temporal gyrus (4) and decreased regional WM volume and fractional anisotropy in the superior temporal gyrus (2). Penhune et al. (3) observed increased GM density in the left motor area (not in the auditory area) and suggested an association with the active use of the dominant sign language. Thus, most studies of deaf adults' brain have stated that GM density is preserved in the auditory cortices (3, 4) but a recent study of hearing-impaired infants showed increased GM density in their left Heschl's gyri (5). These observations led us to ask what would be the brain structures of congenitally deaf adolescents, particularly in their auditory areas.
Magnetic resonance imaging (MRI) could provide information about structural characteristics such as the regional volume, shape, and GM/WM concentrations of individual brains. Voxel-based morphometry (VBM) has been used to measure the regional tissue concentration and volume changes in MRI (7). Furthermore, optimized VBM has been demonstrated to be more accurate than manual delineation despite measuring subcortical structures (8). The recently developed high-dimensional warping algorithm called "Diffeomorphic Anatomical Registration Through Exponentiated Lie Algebra" (DARTEL) (9) could provide high spatial registration accuracy, the most important factor for obtaining good voxel-wise structural analyses.
However, Heschl's gyrus is a variably shaped structure caused by the presence of duplications in the superior temporal gyrus (3), making the normalization of Heschl's gyrus (to standard space) more difficult than the normalization of other cortical areas. Heschl's gyrus appears as one or two gyri (10) and it seems that the right Heschl's gyrus has greater anatomical variability than the left Heschl's gyrus (11). Additionally, previous VBM methods have been criticized for the limited accuracy of their spatial registration (12) compared with the upgraded version (9). Recently, a study compared the accuracy of various registration algorithms in Heschl's gyrus and claimed that the DARTEL algorithm outperformed other registration methods (11). We expected that the newly developed VBM with the DARTEL high-dimensional warping algorithm could reveal additional morphometric information regarding the brains of congenitally deaf adolescents, particularly the auditory cortical areas.
The purpose of the present study was to investigate structural abnormalities of the central auditory systems of congenitally deaf adolescents, including auditory-related sensory areas and subcortical auditory centers. To enhance the accuracy of the present study, we adopted the recently improved VBM8 technique with the DARTEL algorithm for morphometric analyses of the brains of congenitally deaf adolescents using MRI.
We prospectively enrolled eight profoundly congenitally deaf adolescents (mean age ± standard deviation (SD), 15.6 ± 1.19 years; range, 13-17 years; four boys, four girls), who were attending Chuncheon Kyesung Deaf School and nine adolescents with normal hearing who had a similar mean age (15.2±1.09 years; range, 14-18 years; seven boys, two girls). All congenitally deaf adolescents had severe or profound hearing loss (> 85 dB in the better ear; Table 1) and none wore a hearing aid at home or school. They had been using sign language as a major form of communication for at least 7 years and had no difficulty in communication with their classmates and teachers. All normal controls had normal hearing (< 25 dB hearing level at the standard audiological frequency range of 250-8000 Hz). Inclusion criteria for the congenitally deaf adolescents were having profound deafness diagnosed within the first 6 months of life, average or above average intelligence level (about 100 on a non-verbal IQ test) and fluency in Sign Language. The predominant cause of deafness was inherited. The deaf adolescents and normal controls had no history of neurological or psychiatric disease, no history of severe head trauma, and no radiological abnormality as assessed by T1 MRI. All subjects were right handed by the Edinburgh Handedness Inventory.
The present study was approved by the local institutional review board. Written informed consent was obtained from the adolescents' parents or teachers after a thorough explanation of the procedures.
All MR images were scanned using a 1.5-Tesla scanner (Gyroscan ACS-NT; Philips Medical Systems, Best, Netherlands). For consistent head positioning of individuals, pilot coronal, sagittal, and axial turbo-field echo (TFE) T1 MR images were acquired using the following scanning variables: thickness = 10 mm; gap = 10 mm; repetition time/echo time (TR/TE) = 15/5.2 ms; matrix = 256 × 256; field of view (FOV) = 25 × 25 cm; three slices in each orientation; and flip angle = 20°. By the guide of pilot images, coronal three-dimensional T1-weighted TFE MR images were obtained using the following scanning variables: slice thickness = 1.0 mm; no gaps; 160 slices; scanning time = 10 min 13 s; TR/TE/TI = 10/4.3/952 ms; number of signal averages = 1; matrix = 256 × 256; FOV = 22 × 22 cm; and flip angle = 8°, final voxel size = 0.86 × 0.86 × 1.0 mm. Coronal slices were obtained perpendicular to the long axis of the anterior to the posterior commissure in the midsagittal plane. Structural abnormalities revealed by the MR images were screened by a neuroradiologist.
Data were processed and statistically tested using SPM8 (Wellcome Department of Imaging Neuroscience Group, London, UK) and the VBM8 toolbox (http://dbm.neuro.uni-jena.de/vbm) was used for VBM. Data processing was performed on a 64-bit Windows workstation. MR images were bias-corrected and spatially normalized to the DARTEL template by DARTEL high-dimensional spatial normalization (9) with options of "non-linear only" transformations, and then DARTEL was implemented in the VBM8 toolbox. The "non-linear only" option allows the comparison of the absolute tissue amount, which is corrected for individual brain sizes. The accuracy of spatial normalization was visually checked using the Check Registration tool with the DARTEL-normalized T1 MR images of all subjects (Fig. 1). Normalized MR images were segmented into GM, WM, and cerebrospinal fluid (CSF) using an adaptive maximum a posteriori technique that did not require prior information about tissue probabilities. The voxel values of the GM partitions were multiplied by the non-linear components derived only from the normalization matrix to preserve the actual GM values locally (modulation process). Modulated GM smoothed using an 8-mm full-width at half-maximum (FWHM) isotropic Gaussian kernel (IGK). Other parameters were set to default values. The amounts of GM and ICVs were automatically calculated from the GM, WM, and CSF partitions.
ANCOVA controlled for age and gender was applied for analyses of regional volume changes in modulated GM images (13). To exclude edge effects between the boundaries of different tissue types, an absolute threshold masking value of 0.2 was applied. The statistical threshold level was set to false discovery rate (FDR) corrected P < 0.05, and the extent threshold was set to kE > 30 voxels (101 mm3; 1 voxel = 3.375 mm3).
Coordinates were defined by the Montreal Neurological Institute (MNI) coordinate system and the cluster regions were localized with information about their shape, extent, local maxima, based on the standardized template and defined by visual comparison with the atlas of Duvernoy (14). The averaged T1 MR images, created using all the subjects of the present study, were used as the template in the figures and the images are displayed in neurological views.
The mean values of age (normal controls, 15.2 ± 1.09 years; congenitally deaf adolescents, 15.0 ± 2.88 years; P = 0.48, degrees of freedom = 15, SD = 1.03; t-test), gender (P = 0.34; χ2 test), and ICV (normal controls, 1415 ± 132.4 cm3; congenitally deaf adolescents, 1404 ± 112.8 cm3; P = 0.70; F = 0.155; R2 = 0.225; ANCOVA controlled for age and gender) were not different between normal and congenital deaf adolescents. The mean GM volumes between the normal controls and congenitally deaf adolescents were not different (GM: 670 ± 106.3 and 609 ± 60.2 cm3, respectively; P = 0.22; F = 1.71; R2 = 0.569; ANCOVA controlled for age, gender, and ICV). Age was not correlated with GM volume in the correlation or partial correlation analyses (controlled for intracranial volume).
In the auditory centers, the left anterior Heschl's gyrus and both inferior colliculi of congenitally deaf adolescents demonstrated decreased regional GM volumes (Table 2, Fig. 2). These regionally decreased GM volumes were also observed in both nuclei accumbens, the left posterior thalamic reticular nucleus (that wraps much of the thalamus), and both lingual gyri (left dominant) that are known to be the multisensory (auditory-visualsomatosensory) integration area (15). However, increased regional GM volumes were not observed in congenitally deaf adolescents compared with normal controls.
We demonstrated noticeable findings of structural deficits in auditory GM areas and decreased volumes in inferior colliculi, the nucleus accumbens, the left Heschl's gyrus, and the multisensory integrating area of the lingual gyrus, and these results might be the first findings regarding the brain structure of congenitally deaf adolescents. We think that the subjects' age in the present study fits within the age gap between the infant study (5) and the adult studies (1, 2, 3, 4). Functional imaging studies in deaf subjects have revealed auditory cortical activation in response to sign language and other visual stimuli (16, 17, 18, 19). However, our result of decreased GM volume in the left Heschl's gyrus suggests that the neurons of Heschl's gyrus in congenitally deaf adolescents might have been underdeveloped compared with those in normal adolescents, in contrast to previous results obtained from deaf adults. The average age of the subjects in our study was relatively younger (15.2 years) than that in previous morphometric studies (20-30 years) (1, 2, 3, 4). Until adolescence, the normal brain structures change dramatically. In particular, the auditory network of neurofilament-positive axons in the superficial layers IIIb, IIIa, and II mature from 5 years until 11-12 years (20). Thus, in normal individuals, the auditory structures in adolescents could be different from those in adults. The auditory structures of congenitally deaf adolescents could also be different from those in deaf adults or infants because the reorganization of the auditory areas might be slow enough to show various GM volume changes during the neural plastic change and may not reach "normal GM volume" before adulthood. Increased GM volume in the left anterior portion of Heschl's gyrus was reported in hearingimpaired infants aged 12 months (5) and the authors explained that the increased GM volume might come from immature synapses because GM density could decrease with the maturation process of developing children; thus, excessive GM could be interpreted as an immature cortex (21). The immature Heschl's gyrus of deaf infants might show an abnormal development rate and process when we consider that the results of the present study reported decreased GM volume in the left Heschl's gyrus. This relatively late maturation might be explained by functional MRI studies where the superior temporal areas of deafness are functionally stimulated by sign language or other stimuli (16, 17, 18, 19). Furthermore, this plastic change in Heschl's gyrus may occur until adulthood (1). It should be noted, however, that revealed structural differences between infants, adolescents and adults could partially originate due to different techniques used in different studies. It cannot be stated with certainty if all of them are based only on stage of development.
The more variable location and shape of the right Heschl's gyrus (11, 22) might have affected our VBM analyses. We observed a GM volume change only in the left Heschl's gyrus. In addition, the left-side dominance of the temporal lobe's speech area has been considered to be independent of speech or hearing experiences (1, 3). The leftward defect in the posterior left superior temporal gyrus was also reported in a VBM study with deaf adults and the leftward dominance was intact in the same study (4). While their results demonstrated decreased WM volume in the secondary auditory cortex that might represent defects in the auditory-speech area, our results suggest a deficit more directly related to general sound transmission, because the GM volume was found to be decreased in the anterior Heschl's gyrus (the primary auditory cortex) in our study.
Integration of auditory, visual, and somatosensory functions occurs at the early developmental stage of the lingual gyrus (23, 24). The functional role of the lingual gyrus studied in trained musicians was in higher-order harmonic processing, which begins to develop from early childhood (25). Thus, the deficit of the lingual gyrus in congenitally deaf adolescents could be a consequence of the early deprivation of auditory stimuli from various acoustic environments (15, 26).
The inferior colliculus is a major nucleus of the auditory system that receives ascending signals from the lower brainstem nuclei and descending signals from the medial geniculate body, superior colliculus, and auditory cortex. For front-back and up-down sound localization, an abundance of auditory and somatosensory (e.g., trigeminal and cervical) information converges at the inferior colliculus, which functions as a transmission switchboard of multisensory perception (27) and is also believed to be the most metabolically active brain structure (28). The decreased volume of the inferior colliculus could be interpreted as a failure of auditory and somatosensory signals to transit between the auditory and other sensory nuclei.
The present study also revealed decreased GM volume in the subcallosal region, including the nucleus accumbens and thalamic reticular nucleus. The functional role of the subcallosal area is correlated with unpleasant emotions caused by musical dissonance (29) and aversive sounds (30). Additionally, the nucleus accumbens plays an important role in the configuration of adaptive behavioral responses to various environmental sounds (31) and habituation to noise bursts (32). The thalamic reticular nucleus plays a crucial role in controlling auditory thalamo-cortical transmission as an auditory gain controller that modulates the signal intensity to a comfortable level for listening or cancels continuous meaningless background noise (33). The serotonergic axons of the nucleus accumbens stimulate the thalamic reticular nucleus, and serotonin excites the GABAergic neurons of the thalamic reticular nucleus (34). An interconnected circuit also exists between the thalamic reticular nucleus and the nucleus accumbens, with the nucleus accumbens subsequently influencing inhibitory gating on the thalamocortical relay (35). The input of the thalamic reticular nucleus inhibits the neurons of the medial geniculate nucleus (36). The serotonergic neurons of the subcallosal area could modulate transmission from the sensory periphery and brainstem to the cerebral cortex via the thalamic reticular nucleus. The nucleus accumbens?thalamic reticular nucleus system functions by canceling the perception of persistent unpleasant noises (34). The nucleus accumbens and thalamic reticular nucleus are well known auditory gain controllers. Thus, it seems reasonable to think that defects in those structures would be found in profoundly deaf individuals who do not entirely have an input to control its gain at all. In summary, we observed structural deficits of the auditory gain-control system in the thalamus, which controls auditory signal intensity and emotional responses to sounds.
The DARTEL algorithm (9) of SPM8 (adopted in the present study) showed accurate spatial normalization, particularly in the midbrain, Heschl's gyrus, and subcortical structures (12) (Fig. 1). Heschl's gyrus is variably shaped for gyral duplications and the right Heschl's gyrus is anatomically more variable than the left Heschl's gyrus (10). This gyral variability makes the spatial registration of Heschl's gyrus to the template more difficult than other cortical areas. Recently, a validation study of spatial registration was tested for its accuracies in Heschl's gyrus. DARTEL and implicit reference-based registration (IRG) techniques outperformed other registration techniques such as HAMMER, BSpline-based, and unified segmentation based-registration of SPM5 (11). The DICE coefficients of DARTEL and IRG were the best, sensitivity and specificity of DARTEL were second best, after IRG, and positive predictive value was similar between these methods (11). From the marked improvement in spatial registration techniques, we could successfully reveal the structural deficits in the inferior colliculi, Heschl's gyrus, and multisensory areas.
The sample size of the present study was statistically marginal. A confirmative study could be warranted with a larger sample size of congenital deaf adolescents. Technically, the sensitivity and accuracy of VBM may be debatable in measuring subcallosal regions, such as the nucleus accumbens and thalamic reticular nucleus. And other MR Imaging factors could affect the result of VBM (37, 38, 39, 40, 41).
This is the first report regarding the brain structure of congenitally deaf adolescents. Additionally, we first demonstrated noticeable findings of the decreased GM volumes in the auditory cortical and subcortical centers such as inferior colliculi, the nucleus accumbens, left Heschl's gyrus, and the multisensory integrating area of the lingual gyrus. Underdevelopment of primary auditory cortex (Heschl's gyrus) might be related to the loss of general auditory perception. The defects in the nucleus accumbens and thalamic reticular nucleus might explain the incapacity for auditory gain control and failure in the maturation of the auditory-to-limbic connections. The structural abnormalities of those areas in congenital deaf adolescents could be interpreted as neural maldevelopment due to early deprivation of auditory stimuli.
Figures and Tables
Fig. 1
Registration accuracy of inferior colliculi (ICs). The accuracies of spatial normalization in the ICs of normal (a) and congenitally deaf adolescents (b) were checked using the check registration function of SPM8 and the ICs of all subjects were correctly registered. Blue crosshairs represent the same positions in Montreal Neurological Institute space.
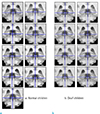
Fig. 2
Decreased regional gray matter volume in congenitally deaf adolescents. The gray matter volumes were regionally decreased in the left Heschl's gyrus (HG), left thalamic reticular nucleus (TRN), bilateral inferior colliculi (ICs), nuclei accumbens (NA), and both lingual gyri (LG) of deaf adolescents. Statistical maps are displayed in neurological views, which were overlaid on averaged T1 templates. Color bar represents T values.
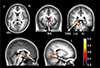
Table 1
Audiometric Test Results
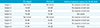
Table 2
Reduced Regional Gray Matter Volume in Congenital Deaf Adolescents
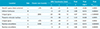
References
1. Emmorey K, Allen JS, Bruss J, Schenker N, Damasio H. A morphometric analysis of auditory brain regions in congenitally deaf adults. Proc Natl Acad Sci U S A. 2003; 100:10049–10054.
2. Kim DJ, Park SY, Kim J, Lee DH, Park HJ. Alterations of white matter diffusion anisotropy in early deafness. Neuroreport. 2009; 20:1032–1036.
3. Penhune VB, Cismaru R, Dorsaint-Pierre R, Petitto LA, Zatorre RJ. The morphometry of auditory cortex in the congenitally deaf measured using MRI. Neuroimage. 2003; 20:1215–1225.
4. Shibata DK. Differences in brain structure in deaf persons on MR imaging studied with voxel-based morphometry. AJNR Am J Neuroradiol. 2007; 28:243–249.
5. Smith KM, Mecoli MD, Altaye M, et al. Morphometric differences in the Heschl's gyrus of hearing impaired and normal hearing infants. Cereb Cortex. 2011; 21:991–998.
6. Morita T, Naito Y, Tsuji J, Nakamura T, Yamaguchi S, Ito J. Relationship between cochlear implant outcome and the diameter of the cochlear nerve depicted on MRI. Acta Otolaryngol Suppl. 2004; 56–59.
7. Good CD, Johnsrude IS, Ashburner J, Henson RN, Friston KJ, Frackowiak RS. A voxel-based morphometric study of ageing in 465 normal adult human brains. Neuroimage. 2001; 14:21–36.
8. Douaud G, Gaura V, Ribeiro MJ, et al. Distribution of grey matter atrophy in Huntington's disease patients: a combined ROI-based and voxel-based morphometric study. Neuroimage. 2006; 32:1562–1575.
9. Ashburner J. A fast diffeomorphic image registration algorithm. Neuroimage. 2007; 38:95–113.
10. Leonard CM, Puranik C, Kuldau JM, Lombardino LJ. Normal variation in the frequency and location of human auditory cortex landmarks Heschl's gyrus: where is it? Cereb Cortex. 1998; 8:397–406.
11. Tahmasebi AM, Abolmaesumi P, Wild C, Johnsrude IS. A validation framework for probabilistic maps using Heschl's gyrus as a model. Neuroimage. 2010; 50:532–544.
12. Ashburner J, Friston KJ. Nonlinear spatial normalization using basis functions. Hum Brain Mapp. 1999; 7:254–266.
13. Friston KJ, Holmes AP, Worsley KJ, Poline JP, Frith CD, Frackowiak RSJ. Statistical parametric maps in functional imaging: a general linear approach. Hum Brain Mapping. 1995; 2:189–210.
14. Duvernoy HM. The human brain: surface, three-dimensional sectional anatomy and MRI. New York: Springer-Verlag;1999.
15. Falchier A, Clavagnier S, Barone P, Kennedy H. Anatomical evidence of multimodal integration in primate striate cortex. J Neurosci. 2002; 22:5749–5759.
16. Campbell R, MacSweeney M, Waters D. Sign language and the brain: a review. J Deaf Stud Deaf Educ. 2008; 13:3–20.
17. Capek CM, Woll B, MacSweeney M, et al. Superior temporal activation as a function of linguistic knowledge: insights from deaf native signers who speechread. Brain Lang. 2010; 112:129–134.
18. Finney EM, Fine I, Dobkins KR. Visual stimuli activate auditory cortex in the deaf. Nat Neurosci. 2001; 4:1171–1173.
19. Neville HJ, Bavelier D, Corina D, et al. Cerebral organization for language in deaf and hearing subjects: biological constraints and effects of experience. Proc Natl Acad Sci U S A. 1998; 95:922–929.
20. Moore JK, Guan YL. Cytoarchitectural and axonal maturation in human auditory cortex. J Assoc Res Otolaryngol. 2001; 2:297–311.
21. Gogtay N, Giedd JN, Lusk L, et al. Dynamic mapping of human cortical development during childhood through early adulthood. Proc Natl Acad Sci U S A. 2004; 101:8174–8179.
22. Abdul-Kareem IA, Sluming V. Heschl gyrus and its included primary auditory cortex: structural MRI studies in healthy and diseased subjects. J Magn Reson Imaging. 2008; 28:287–299.
23. Giard MH, Peronnet F. Auditory-visual integration during multimodal object recognition in humans: a behavioral and electrophysiological study. J Cogn Neurosci. 1999; 11:473–490.
24. Macaluso E, Frith CD, Driver J. Modulation of human visual cortex by crossmodal spatial attention. Science. 2000; 289:1206–1208.
25. Schmithorst VJ, Holland SK. The effect of musical training on the neural correlates of math processing: a functional magnetic resonance imaging study in humans. Neurosci Lett. 2004; 354:193–196.
26. Bavelier D, Neville HJ. Cross-modal plasticity: where and how? Nat Rev Neurosci. 2002; 3:443–452.
27. Shore SE. Auditory/somatosensory interactions. In : Squire LR, editor. Encyclopedia of neuroscience. San Diego: Academic Press;2009. p. 691–695.
28. Sokoloff L, Reivich M, Kennedy C, et al. The [14C] deoxyglucose method for the measurement of local cerebral glucose utilization: theory, procedure, and normal values in the conscious and anesthetized albino rat. J Neurochem. 1977; 28:897–916.
29. Blood AJ, Zatorre RJ, Bermudez P, Evans AC. Emotional responses to pleasant and unpleasant music correlate with activity in paralimbic brain regions. Nat Neurosci. 1999; 2:382–387.
30. Zald DH, Pardo JV. The neural correlates of aversive auditory stimulation. Neuroimage. 2002; 16:746–753.
31. Muhlau M, Rauschecker JP, Oestreicher E, et al. Structural brain changes in tinnitus. Cereb Cortex. 2006; 16:1283–1288.
32. McCullough LD, Sokolowski JD, Salamone JD. A neurochemical and behavioral investigation of the involvement of nucleus accumbens dopamine in instrumental avoidance. Neuroscience. 1993; 52:919–925.
33. Jones EG. The thalamus. 2nd ed. Cambridge, UK: Cambridge University Press;2007.
34. Rauschecker JP, Leaver AM, Muhlau M. Tuning out the noise: limbic-auditory interactions in tinnitus. Neuron. 2010; 66:819–826.
35. O'Donnell P, Lavin A, Enquist LW, Grace AA, Card JP. Interconnected parallel circuits between rat nucleus accumbens and thalamus revealed by retrograde transynaptic transport of pseudorabies virus. J Neurosci. 1997; 17:2143–2167.
36. Yu XJ, Xu XX, Chen X, He S, He J. Slow recovery from excitation of thalamic reticular nucleus neurons. J Neurophysiol. 2009; 101:980–987.
37. Kim JH, Kim SH, Shin HS, et al. A study of changes of inversion time effect on brain volume of normal volunteers. J Korean Soc Magn Reson Med. 2013; 17:286–293.
38. Jung WB, Kang MJ, Son DB, et al. Reproducibility analysis of brain volumetry measured from inter MR scanner of multi-institute. J Korean Soc Magn Reson Med. 2012; 16:243–252.
39. Jung WB, Son DB, Kim YJ, Kim YH, Eun CK, Mun CW. A comparison study on human brain volume of white matter, gray matter and hippocampus depending on magnetic resonance imaging conditions and applied brain template. J Korean Soc Magn Reson Med. 2011; 15:242–250.
40. Choi N, Nam Y, Kim DH. Cortical thickness estimation using DIR imaging with GRAPPA factor 2. J Korean Soc Magn Reson Med. 2010; 14:56–63.
41. Kim HD, Chang KH, Han MH, Kim HJ, Lee SG, Lee MC. The significance and limitation of MR volumetry: comparison between normal adults and the patients with epilepsy and hippocampal sclerosis. J Korean Soc Magn Reson Med. 2002; 6:47–54.