Abstract
Adjuvants can be defined as pharmacological and immunological components that are able to modify and/or enhance antigen-specific immune responses. Based on the interdisciplinary research between immunology and material science/engineering, various vaccine adjuvant materials have been developed. By rational design and engineering of antigen or adjuvant materials, immune-modulatory vaccine systems generated to activate immune system. Here, we review the current progress of bioengineered prophylactic and/or therapeutic vaccine adjuvant for cancer and/or infectious disease, and discuss the prospect of future vaccine adjuvant materials.
Adjuvants materials are compounds or macromolecular complexes that can enhance the potency and longevity of antigen-specific immune response [1,2,3,4]. By the combination of adjuvants with vaccine antigen, the original immunogenicity of antigens can be modulated for appropriate immune responses, improvement of vaccine efficacy and reduction of the amount of antigen or number of immunizations required [3,4,5,6,7,8,9]. Although vaccines based on inactivated viruses or bacteria are sufficiently immunogenic, adjuvant materials are still widely adopted to enhance the immune response. Especially, various adjuvant materials have been adopted to elicit protective antibody responses in recombinant protein antigen-based vaccine system which shows low immunogenicity (Table 1). In general, adjuvants can be classified into two categories according to their component sources, physiochemical properties or mechanisms of action [2,3]: 1) immunostimulants that intrinsically act on the immune system to enhance immune responses of various antigens. Typical examples are Toll-like receptor (TLR) ligands, cytokines, saponins and bacterial exotoxins which stimulate immune responses; 2) delivery carriers that deliver and present vaccine antigens to the antigen presenting cells with a controlled manner to induce adaptive immune response and increase the antigen-specific immune response. In this case, the delivery carrier can also deliver the immunostimulants simultaneously. This category contains various polymer microspheres and immune stimulating complexes, emulsions (oil-in-water or water-in-oil), aluminum salts, liposomes, and virosomes [1,6,7,8,9].
When purified protein antigens were immunized, modest antibody response with little or no T-cell response were generally induced and multiple injections are required to elicit sufficient antibody responses [2,3]. Immunologist and vaccine users also need vaccine adjuvant materials that can enhance the efficacy of low immunogenic antigens and induce appropriate immune responses for long time (Table 2). The major roles of vaccine adjuvant materials can be discussed in five points of view [8,9,10,11]. The first role of vaccine adjuvant is dose sparing. According to the recent report, it was estimated that approximately 1 billion doses of the vaccine could be produced in pandemic condition, which is insufficient to cover the worldwide population. Therefore, to increase global vaccine supply, both the expansion of vaccine technologies beyond egg-based production to include recombinant vaccines and the use of adjuvant materials were highly suggested. As mentioned, any recombinant protein antigen-based vaccines are low immunogenic, although they have advantages in manufacturing points of view. In this context, the amount of recombinant pandemic influenza protein antigens can be reduced by combining the antigen with various adjuvant materials. The second one is the capability of more rapid immune response. In a specialized application such as biodefense vaccines for pandemic flu, anthrax and other potential bioterrorism weapons, a single-shot vaccine is highly required. By adding suitable adjuvant materials, the immune response was accelerated and excludes additional vaccination. The third one is the broadeing of antibody response. The substantial antigenic drift and/or strain variations of influenza viruses are one of the big hurrdles in the development of universal vaccine. These pathogens also include human immunodeficiency virus, human papillomavirus, and malaria parasite. In this field, the capability of vaccine adjuvant materials that can broaden an immune response profile is essential. The fourth one is the tuning of magnitude and functionality of antibody response. Although aluminum salts or oil-in-water emulsions are known to induce a greater magnitude of antibody responses to various vaccine antigens, it still require vaccine adjuvant materials that can increase overall antibody titer as well as a number of functional antibodies which has higher affinity for vaccine antigens. The fifth one is the induction of effective T-cell response. Unfortunately, the commonly used two adjuvant materials approved for human use (aluminum salts and oil-in-water emulsion-based adjuvants) are not effective in T-cell response [11,12,13]. Therefore, new vaccine adjuvant material that can elicit more effective engagement of T helper cells for optimizing the quality and durability of antibody responses or induce effector CD4+ or CD8+ T cells for the clearance intracellular pathogens, are highly required (Table 2). In fact, the fifth role of vaccine adjuvant is particularly important in the development of vaccines against pathogens that are controlled by cellular immune responses (malaria, tuberculosis and leishmaniasis) [5].
The desired immune response in cancer therapy involves the activation of interferon γ (IFN-γ) producing type 1 T helper cells (Th1) and cytotoxic T lymphocytes (CTLs) [14,15,16,17]. Although aluminum salt-based vaccine adjuvant has been widely used as prophylactic vaccines which promotes type 2 helper T cells (Th2)-dependent immunity, it was not effective for the induction of strong Th1-dependent immunity. Because the immune system recognizes pathogen-associated molecular patterns by means of pathogen-recognition receptors such as TLRs, various adjuvant materials that can stimulate the innate immunity have been adopted in cancer immunotherapy (Tables 1, 2). Although several TLR ligands show significant promise for the treatment of cancer, the interest is centered on the potential use them to complement conventional cancer therapeutic modalities such as radiation, monoclonal antibodies and/or cytotoxic drugs [14,15]. The stimulation of immune system and induction of significant antitumor therapeutic effect of TLR may be explained by the four principal mechanisms [14,16,17,18]. First, the antitumor activity through TLR can be achieved by the activation of natural killer cells, monocytes and macrophages as well as the induction of cytokines with direct or indirect antitumor activities (IFN-α, IFN-γ, and tumor necrosis factor α). Second, the helper T-cell responses of CD4 (Th1) and CD8 (CTL) against tumor antigens can be stimulated by antigen release from tumor cells killed either by innate mechanisms or by co-administrated cytotoxic agents. Third, antibody-dependent effector functions can be enhanced by stimulation through TLR9 and possibly other TLR. For example, anti-CD20 requires antibody-dependent cellular cytotoxicity for optimal killing of tumor cells. Fourth, it can induce apoptosis in TLR-positive tumors. For example, in TLR9+ chronic lymphocytic leukemia cells 75 and TLR3+ breast carcinoma cells 76, apoptosis in vitro was efficiently induced. In fact, the evidence for the first three mechanisms was provided through extensive studies of TLR9 ligands in rodent models of cancer. In addition, another TLR-based approach to cancer is the use of TLR as adjuvants for therapeutic vaccination against tumor-associated antigens [14,15].
Because prophylactic vaccines play an important role in preventing a variety of infectious diseases, various vaccine adjuvants that can increase the effectiveness of vaccine have been developed and some of them are already in clinics (Table 1) [10]. As an alternative to conventional inactivated or live-attenuated pathogen based antigens which show side effect and toxicity, subunit vaccine antigens were highly interested with recent advances in genomics and proteomics. Several new approaches to develop universal influenza vaccines that can induce broadly cross-protective immunity against conserved antigenic targets such as the hemagglutinin stalk domain and the extracellular domain of M2 (M2e), have been also developed [7]. However, such subunit vaccines are in general less immunogenic than pathogen-based antigen and often require the adjuvant to achieve protective immune responses. Until now, a few vaccine adjuvants have been licensed for prophylactic vaccine in human. Aluminum salts has been extensively used for more than 70 years and was the only adjuvant approved in the United States. Oil-in-water emulsions, such as MF59, is licensed in Europe as adjuvant materials for influenza vaccines. A combination adjuvant system that contains monophosphoryl lipid A (MPL) adsorbed to aluminum salts (AS04) is approved for hepatitis B virus and human papillomavirus vaccines in Europe and has been recently licensed in the United States. Among various TLR agonists, TLR3, TLR7, TLR8, and TLR9 have shown promise as treatments for infectious diseases, especially viral infections [14,19,20,21,22,23,24,25,26]. The main mechanisms of antiviral activity through TLR are the induction of type I IFNs and IFN-dependent antiviral effect, the enhancement of natural killer cell cytotoxicity, and virus-specific T-cell responses [20,21,22,23,24,25,26]. After imiquimod has been approved for treatment of genital warts caused by human papillomavirus, TLR7 agonists have been intensively studied [23]. TLR3 agonists have been also highly interested due to their clinical potential in human immunodeficiency virus (HIV). It was also reported that the prophylactic treatment with TLR9 agonist showed enhanced resistance to a wide range of viral and bacterial diseases.
In summary, the immunomodulatory materials that have been designed and engineered for antigen-specific immune responses are expected as promising vaccine adjuvants both for cancer immunotherapy and control of infectious disease. The optimal combination of antigens, adjuvants and delivery carriers is very important to maximize the effectiveness both in cancer immunotherapy and infectious disease control [6,27]. Several issues should be overcome to meet the demands for new adjuvants [2,3]. Especially, possible side effects and toxicity still remain and these issues are particularly important for the development of pediatric vaccines. Up to now, the vaccine adjuvants licensed for human vaccines in the United States and/or Europe include aluminum salts, oil-in-water emulsions, virosomes and AS04 (MPL preparation with aluminum salts). Although vaccine adjuvants designed for therapeutic uses, such as in cancer, may have more tolerance in evaluating the side effect if the therapeutic effect is excellent, the choice of well-defined immunostimulatory component or formulations that can enhance optimum immune response without compromising safety is essential in the approval for clinics and subsequent commercialization [10,11]. Any single immunostimulants or delivery system will not be sufficient to induce both broad and long-lasting immunity (Tables 1, 2). In addition, the next generation of recombinant vaccines against malaria, tuberculosis and HIV and/or acquired immune deficiency syndrome, will require very strong and long-lasting antibody responses as well as potent cell-mediated immunity based on CD4 and CD8 T-cell responses [5,11]. Therefore, the future effective adjuvant systems can be developed by combining one or more immunostimulants and delivery system. It should be also emphasized that the adjuvant effects can vary according to immunization dose, schedule, route of administration, status of host, and target antigens. Since most of the pathogen gain access inside the body of host by mucosal routes, administration of vaccine for adequate localised immune response is very important [28,29]. In this respect, bioengineered adjuvant materials having mucoadhesive properties enable close contact with mucosal tissues and enhance the residence time, mucosal uptake, thereby increase the bioavailability of the antigen, resulting in enhanced mucosal immunity.
Figures and Tables
Table 1
Adjuvant materials approved for human vaccines
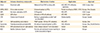
Alum, aluminum salts; HAV, hepatitis A virus; HBV, hepatitis B virus; HPV, human papillomavirus; APCs, antigen presenting cells; MPL, monophosphoryl lipid A; LPS, lipopolysaccharides; TLR, Toll-like receptor; NF-kB, nuclear factor kB; VLP, virus-like particles; PAMP, pathogen-associated molecular pattern; HA, hemagglutinin.
Table 2
Selected human vaccine adjuvant candidates under clinical development
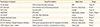
Notes
References
1. Hubbell JA, Thomas SN, Swartz MA. Materials engineering for immunomodulation. Nature. 2009; 462:449–460.


3. Petrovsky N, Aguilar JC. Vaccine adjuvants: current state and future trends. Immunol Cell Biol. 2004; 82:488–496.


4. Reed SG, Bertholet S, Coler RN, Friede M. New horizons in adjuvants for vaccine development. Trends Immunol. 2009; 30:23–32.


5. Griffiths KL, Khader SA. Novel vaccine approaches for protection against intracellular pathogens. Curr Opin Immunol. 2014; 28:58–63.


6. Correia-Pinto JF, Csaba N, Alonso MJ. Vaccine delivery carriers: insights and future perspectives. Int J Pharm. 2013; 440:27–38.


7. Kang SM, Kim MC, Compans RW. Virus-like particles as universal influenza vaccines. Expert Rev Vaccines. 2012; 11:995–1007.


8. Irvine DJ, Swartz MA, Szeto GL. Engineering synthetic vaccines using cues from natural immunity. Nat Mater. 2013; 12:978–990.


9. Moon JJ, Huang B, Irvine DJ. Engineering nano- and microparticles to tune immunity. Adv Mater. 2012; 24:3724–3746.


10. Mbow ML, De Gregorio E, Valiante NM, Rappuoli R. New adjuvants for human vaccines. Curr Opin Immunol. 2010; 22:411–416.


11. Riese P, Schulze K, Ebensen T, Prochnow B, Guzman CA. Vaccine adjuvants: key tools for innovative vaccine design. Curr Top Med Chem. 2013; 13:2562–2580.


12. Galli G, Hancock K, Hoschler K, et al. Fast rise of broadly cross-reactive antibodies after boosting long-lived human memory B cells primed by an MF59 adjuvanted prepandemic vaccine. Proc Natl Acad Sci U S A. 2009; 106:7962–7967.


13. Khurana S, Verma N, Yewdell JW, et al. MF59 adjuvant enhances diversity and affinity of antibody-mediated immune response to pandemic influenza vaccines. Sci Transl Med. 2011; 3:85ra48.


14. Kanzler H, Barrat FJ, Hessel EM, Coffman RL. Therapeutic targeting of innate immunity with Toll-like receptor agonists and antagonists. Nat Med. 2007; 13:552–559.


15. Wiley SR, Raman VS, Desbien A, et al. Targeting TLRs expands the antibody repertoire in response to a malaria vaccine. Sci Transl Med. 2011; 3:93ra69.


16. Draper E, Bissett SL, Howell-Jones R, et al. A randomized, observer-blinded immunogenicity trial of Cervarix((R)) and Gardasil((R)) human papillomavirus vaccines in 12-15 year old girls. PLoS One. 2013; 8:e61825.
18. Honda K, Takaoka A, Taniguchi T. Type I interferon [corrected] gene induction by the interferon regulatory factor family of transcription factors. Immunity. 2006; 25:349–360.


19. Iwasaki A, Medzhitov R. Toll-like receptor control of the adaptive immune responses. Nat Immunol. 2004; 5:987–995.


21. Sansonetti PJ. The innate signaling of dangers and the dangers of innate signaling. Nat Immunol. 2006; 7:1237–1242.


22. Akira S, Uematsu S, Takeuchi O. Pathogen recognition and innate immunity. Cell. 2006; 124:783–801.


23. Chang YC, Madkan V, Cook-Norris R, Sra K, Tyring S. Current and potential uses of imiquimod. South Med J. 2005; 98:914–920.


24. Salaun B, Coste I, Rissoan MC, Lebecque SJ, Renno T. TLR3 can directly trigger apoptosis in human cancer cells. J Immunol. 2006; 176:4894–4901.


25. Jahrsdorfer B, Wooldridge JE, Blackwell SE, et al. Immunostimulatory oligodeoxynucleotides induce apoptosis of B cell chronic lymphocytic leukemia cells. J Leukoc Biol. 2005; 77:378–387.


26. Klinman DM. Immunotherapeutic uses of CpG oligodeoxynucleotides. Nat Rev Immunol. 2004; 4:249–258.

