Abstract
PURPOSE
This study aimed to create a digitized database of fabricated obturators to be kept for patients' potential emergency needs.
MATERIALS AND METHODS
A chairside intraoral scanner was used to scan the surfaces of an acrylic resin obturator. The scanned data was recorded and saved as a single standard tessellation language file using a three-dimensional modeling software. A simulated obturator model was manufactured using fused deposition modeling technique in a three-dimensional printer.
During rehabilitation, maxillectomy patients are treated with obturators, which are essential for oral functions such as speech, swallowing, mastication, and esthetics.123 Loss of or damage to an obturator results in both functional disability and cosmetic disfigurement. Conventional fabrication of an obturator is complex and needs multiple scheduled visits, and an alternative process is needed for rapid fabrication in emergency cases, such as disaster-related damage or loss. Digital technology is creating exciting opportunities for improving the delivery of maxillofacial prostheses.4 Digitized data for any object can be obtained from various resources, such as computerized tomography (CT) imaging, three-dimensional (3D) photography, and 3D scanning. Intraoral scanners are among the 3D scanning tools that can be used to directly obtain 3D models of the mouth. These scanners record the geometry of the object by measuring the distance between the tip of scanner's sensor and the target object by utilizing different technologies to convert the optical data to a 3D model. Lava COS is a 3D scanner and uses a 3D video system comprising active wavefront sampling that captures 20 3D frames per second, resulting in a high-resolution final model.5
Construction of a 3D model can be performed using surface-based recording, as it is commonly used in various types of 3D modeling software. This model does not contain any volumetric data but instead uses a triangle-based mesh representation of the 3D surface geometry. Standard Tessellation Language (STL) is an open-source surface-based format, which is easily accessible through most commercial software applications. Surface models using such a method have been widely used for rapid prototyping and computer-aided manufacturing. These datasets allow for easy information exchange and communication among users. STL data can be saved in two different formats, American Standard Code for Information Interchange (ASCII) and binary.6 The ASCII format is less commonly used due to the large size of the resultant file, but it has an advantage in that it can be easily modified for debugging purposes. The binary format is more complicated in its syntax, but it generates smaller file sizes and is therefore used more often. The development of 3D printing technologies has allowed for the accurate printing of the complex shapes of maxillofacial prosthetics with details including the precise simulation of the undercut areas.7 These technologies utilize an additive process of building an object geometry in layers from a virtually sectioned 3D model. Fused deposition modeling (FDM) is one such technology, in which a thermoplastic material is extruded from a nozzle layer by layer, controlled by temperature difference.8 This technique has been successfully used for the fabrication of maxillofacial prosthetics for over the past two decades.9
Assessing the accuracy of 3D models can be done by linear or 3D measurements. In the case of 3D assessment, the scan of an object's geometric form is compared to the original and the difference between the two forms is taken as the scanning accuracy. The working concept of intraoral scanners involves merging multiple 3D images scanned with different angles to build the final 3D model. This process may possibly lead to recording errors of varying degrees, which depend on the scanning technology and the recording algorithms used.10 The accuracy of intraoral scanners has been tested and verified in many published studies.11121314
Using 3D models of fabricated obturators that have been saved in a digitized database will enable the rapid restoration of the basic oral function and appearance provided by an obturator while avoiding time-consuming conventional fabrication methods. This study aimed for and successfully provided a proof-of-concept of a digitized database of fabricated obturators at the Maxillofacial Prosthetics Clinic of Tokyo Medical and Dental University, to be kept for patients' potential emergency needs.
The creation of the digitized database was initiated by creating a 3D model of a patient's and using this model as a basis for 3D printing from an appropriate material for a hypothetical emergency case. The flowchart of the digitized database process is detailed in (Fig. 1).
An acrylic resin obturator for edentulous maxillectomy patients with a open hollowed bulb was sprayed with titanium oxide powder (Lava Powder; 3D Espe, St. Paul, MN, USA), recording the 3D surface sections during scanning, according to the manufacturer's instructions for accurate 3D scanning (Fig. 2). The scanning was performed using a chairside intraoral scanner (Lava COS; 3D Espe, St. Paul, MN, USA). The Lava scanning protocol was done, consisting of calibration using a small calibration block followed by scanning of the obturator surfaces according to an arbitrary scan path. Four scans were performed with a 10-min interval between the scans, with two scans of the obturator's polished surfaces and the other two of the fitting surfaces. The scanning time was 7 min (the maximum time limit) for each scan. The scanned data was checked for surface scanning quality on the Lava COS monitor (Fig. 3). After the scanning and the preliminary evaluation of surface scanning quality, the scan files were sent to the scanner provider, as a post-processing cycle was needed to recalculate the recorded surfaces and compensate for potential errors. Ultimately, a high-resolution model was obtained.
The scanned data was saved as binary STL files, which were imported into 3D modeling software (Artec 3D Studio; Artec, Palo Alto, CA, USA). The files were compiled into a single STL file representing surface shape to provide the data for the combined surfaces of the obturator. Small artifacts such as holes and/or triangulation errors were repaired according to the modeling software's built-in algorithm. The final STL file was evaluated for 3D printing suitability using a 3D printing software (Netfabb Studio Basic; netfabb GmbH, Lupburg, Germany).
Three-dimensional printing, comprising an automatic production technique guided by a digital 3D model, is a suitable technology for manufacturing complex geometric shapes. Obturators have complex geometry that necessitates a layer-by-layer printing technique; therefore, fused deposition modeling was selected for 3D printing. A digitized 3D obturator model was manufactured using acrylonitrile butadiene styrene (Portabee Go; Romscraj, Singapore) on a 3D printer (Portabee Go) (Fig. 4). The obturator was manufactured on the building platform of the 3D printer layer-by-layer; once a layer was light-cured, a new layer of resin was applied until the obturator was completely constructed. The print nozzle was 0.4 mm and the layer thickness was set to 0.1 mm. The working dimensions on the platform were 120 cm for X, 168 mm for Y, and 135 mm for Z. The obturator was cleaned and any excess supporting structures were removed from the surface as they were deposited in thin and brittle walls.
Accuracy assessments of 3D models were performed in 3D geometry evaluation. For this purpose, the original obturator was scanned using a cone-beam CT scanner (3DX multi-image micro CT FPD, Morita, Japan), and the scanned obturator was saved as a digital imaging and communication in medicine (DICOM) file format. DICOM data was imported into 3D modeling software Mimics 11.11 (Materialise NV, Belgium) to produce a virtual 3D reference model and saved as a binary STL file. A similar method was used to scan the manufactured obturator for comparison. The original CT scanned obturator (Reference scan model), the original Lava scanned obturator (Scan1 model), and the manufactured CT scanned obturator (Scan2 model) were geometrically evaluated using a computer aided testing (CAT) software (SpGauge, Armonicos Co., Ltd., Japan) with an error scale of 1.0 mm. The geometry of the scan1 model was compared with the geometry of the reference scan model. The geometry of the scan2 model was also compared with the geometry of the reference scan model.
The entire surface of the obturator was successfully scanned regardless of its structural complexity, modeled as 3D data, and stored in the maxillofacial prosthetics clinic digital system in a relatively small file size (19.6 MB). The model was used to successfully manufacture an obturator that had accurate dimensions compared to the original. Fig. 5 compares the geometries of the original CT scanned obturator and the original Lava scanned obturator. Fig. 6 compares the geometries of the original CT scanned obturator and the manufactured CT scanned obturator.
Provision of obturators remains the most frequent treatment option worldwide for maxillectomy. Obturators have become especially important in complicated emergency cases, such as disaster-related maxillofacial damage or loss of obturators. In such situations, conventional fabrication of obturators is not rapid enough. Therefore, we aimed to show the feasibility of an alternative method to produce these prostheses more rapidly from a digitized database for rehabilitation of patients in an emergency situation. Conventionally, fabrication of an obturator is a complex task that requires a highly skilled maxillofacial prosthodontist and a dental laboratory technician. Therefore, there is an approximately five-week long lead time before the patient is able to use the obturator. Modern digital technology, including 3D scanning and printing, opens up the possibility of manufacturing maxillofacial prostheses more efficiently and with shorter lead time.15
The advantage of using a chairside intraoral scanner is apparent in that the obturator can be scanned while the patient is sitting in the dental chair and the scanner allows a shorter scanning time; furthermore, the patient can walk out the clinic with the obturator. Additionally, the scanner monitor allows for immediate evaluation of scanning quality. However, multiple scans were needed for the surfaces of the obturator in order to combine the images and achieve a higher accuracy. The scanning time was also limited to 7 min, which made it impossible to scan the entire obturator surface in a single scanning session. As a consequence, 4 scanning sessions over a total of 38 min were necessary. Three-dimensional printing technology allows for the production of objects with complex 3D geometry. In this study, an obturator, one such complex object, could be accurately printed out from a scanned model using fused deposition modeling technique.
Evidence of the clinical applicability of the re-fabricated obturators is limited to our current experimental results, and further studies are needed. However, 3D printing of a virtual model and its individual testing usually lead to further developmental treatment phases before further clinical experience can be obtained. In addition, as the obturator in this study was a proof-of-concept, the manufacturing material used was not a standard dental material. Consequently, more studies using 3D printable dental materials are needed. Furthermore, the material for supporting structures in this study was non-soluble, but it may be better to use a soluble material that can make the removal of material more efficient and hands-free. Like other 3D printing technologies, fused deposition modeling has some disadvantages that include: 1) laying out multiple layers often leads to seams which can be seen as lines between each layer, andan extra procedure of finishing and polishing can be added to remove these lines 2) support structures are required for complex geometrical objects, which can lead to difficulty in removing them, and a soluble material can be used to solve it. In the future, further studies will be needed to validate the feasibility of the presented method in a case series.
This study provides a proof-of-concept for the use of digital technology to create a digitized database of obturators for edentulous maxillectomy patients. Such a database could provide an advanced technological solution for the rapid rehabilitation of maxillectomy patients in disaster-related emergency cases.
Figures and Tables
Fig. 2
Acrylic resin obturator sprayed with titanium oxide powder. (A) Polished surfaces, (B) Fitting surfaces.
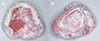
References
1. Elbashti ME, Hattori M, Sumita YI, Taniguchi H. Evaluation of articulation simulation system using artificial maxillectomy models. J Oral Rehabil. 2015; 42:678–684.
2. Sumita YI, Ozawa S, Mukohyama H, Ueno T, Ohyama T, Taniguchi H. Digital acoustic analysis of five vowels in maxillectomy patients. J Oral Rehabil. 2002; 29:649–656.
3. Kumar P, Alvi HA, Rao J, Singh BP, Jurel SK, Kumar L, Aggarwal H. Assessment of the quality of life in maxillectomy patients: A longitudinal study. J Adv Prosthodont. 2013; 5:29–35.
4. van Noort R. The future of dental devices is digital. Dent Mater. 2012; 28:3–12.
5. Syrek A, Reich G, Ranftl D, Klein C, Cerny B, Brodesser J. Clinical evaluation of all-ceramic crowns fabricated from intraoral digital impressions based on the principle of active wavefront sampling. J Dent. 2010; 38:553–559.
6. Gibson I, Rosen D, Stucker B. Additive manufacturing technologies: 3D printing, rapid prototyping, and direct digital manufacturing. 2nd ed. New York: Springer;2014.
7. Lethaus B, Lie N, de Beer F, Kessler P, de Baat C, Verdonck HW. Surgical and prosthetic reconsiderations in patients with maxillectomy. J Oral Rehabil. 2010; 37:138–142.
8. Rimell JT, Marquis PM. Selective laser sintering of ultra high molecular weight polyethylene for clinical applications. J Biomed Mater Res. 2000; 53:414–420.
9. Sabol JV, Grant GT, Liacouras P, Rouse S. Digital image capture and rapid prototyping of the maxillofacial defect. J Prosthodont. 2011; 20:310–314.
10. Chen Y, Medioni G. Object modeling by registration of multiple range images. Image Vis Comput. 1992; 14:145–155.
11. Luthardt RG, Loos R, Quaas S. Accuracy of intraoral data acquisition in comparison to the conventional impression. Int J Comput Dent. 2005; 8:283–294.
12. Mehl A, Ender A, Mörmann W, Attin T. Accuracy testing of a new intraoral 3D camera. Int J Comput Dent. 2009; 12:11–28.
13. Ender A, Mehl A. Full arch scans: conventional versus digital impressions-an in-vitro study. Int J Comput Dent. 2011; 14:11–21.
14. van der Meer WJ, Andriessen FS, Wismeijer D, Ren Y. Application of intra-oral dental scanners in the digital workflow of implantology. PLoS One. 2012; 7:e43312.
15. Sykes LM, Parrott AM, Owen CP, Snaddon DR. Applications of rapid prototyping technology in maxillofacial prosthetics. Int J Prosthodont. 2004; 17:454–459.