Abstract
Implant success is achieved by the synergistic combination of numerous biomechanical factors. This report examines the mechanical aspect of implants. In particular, it is focused on macrodesign such as thread shape, pitch, width and depth, and crestal module of implants. This study reviews the literature regarding the effect of implant thread geometry on primary stability and osseointegration under immediate loading. The search strategy included both in vitro and in vivo studies published in the MEDLINE database from January 2000 to June 2014. Various geometrical parameters are analyzed to evaluate their significance for optimal stress distribution, implant surface area, and bone remodeling responses during the process of osseointegration.
Dental implants have gained popularity as a successful treatment modality for restoring edentulism. Many longterm implant studies have revealed their survival rates exceeding 95%.1,2,3 However, the potential for clinical failures and limiting factors is still present as a great concern for clinicians. Traditionally, a healing period of 3 to 6 months has been recommended for osseointegration after implant placement. Despite high predictability of this traditional protocol, a long, unloaded period is a major drawback especially for patients with strong esthetic demand. Even if patients have decent provisional prostheses fabricated, they must undergo a series of follow-up appointments for adjustments and maintenance. Therefore, considerable scientific interest has been focused on reducing the healing period and loading of the implant.4
The definitions of immediate loading vary based on its indications and protocols.5,6 Immediate loading frequently refers to the placement of a prosthesis in occlusion within 48 hours after implant surgery.7 However, prosthesis can often be fabricated immediately after the surgery to restore a single tooth or partially edentulous dentition. This protocol may show a significant advantage to both clinician and patient. Previous studies on immediate loading have reported survival rates comparable to conventional loading.8,9,10 However, the extent of loading and primary stability required for successful osseointegration under immediate loading is still open to debate and it is necessary to set standardized protocol guidelines.
The presence of osseointegration is critical to evaluate implant success. The effective bond between an implant and its surrounding bone is created by various mechanical factors. One of the key factors is implant design since it determines primary stability and stress distribution during osseointegration. The geometric features of an implant influence sufficient initial contact to facilitate primary stability of the implant.11 It also plays an important role on implant capacity to withstand forces during the process of osseointegration. Therefore, the optimal implant design itself can improve the potential osseointegration process and the primary and secondary stability of the implant.
There are two types of the implant design; macrodesign and microdesign. The former includes thread geometry and body shape while the latter consists of implant material and surface treatment and morphology.12 Dental implants on the market today are available in many different thread patterns. In particular, this literature review was focused on thread geometry such as shape, pitch, width and depth, and crestal module as described in Fig. 1. The purpose of this report sought to evaluate the effect of different implant thread designs on load distribution and primary stability at the implant-bone interface in an immediate loading situation.
This study reviews the literature dealing with the influence of implant thread geometry on primary stability of osseointegrated implants under immediate loading. A MEDLINE search was conducted using the PubMed search engine. The keywords used included; implant thread, implant macrodesign, immediate loading, thread geometry, thread shape, thread pitch, thread depth, thread width, microthreads, crest module and combinations of these keywords. The articles published from January 2000 to June 2014 were accepted. Both in vitro and in vivo studies were included in this review.
Most dental implants can be found in various thread shapes developed for effective inserting and force transmission. Threaded implants are inserted into the osteotomy site by creating linear motion through rotation. Thread shapes available for screw-retained implants include square shape, V-shape, buttress and reverse buttress threads, which are defined by the thread thickness and face angle.13
Once an implant is inserted, bone undergoes constant remodeling against external stress, called bone homeostasis. When an implant receives optimal functional load, the surrounding bone experiences remodeling and produces woven bone. However, under extreme adverse stresses, microfractures occur in the alveolar bone inducing "osteoclastogenesis". 14 Since bone formation is not fast enough to fill in the damage, the defect becomes worse, resulting in severe bone loss and ultimately implant failure.15,16 However, the optimal stress distribution is difficult to achieve, and too little or much stress can induce bone resorption.14,17 Therefore, implant threads should be fabricated to increase surface contact area and favorable forces while reducing adverse stimuli.
Recently, the finite element analysis (FEA) has been utilized to understand the effect of those geometric parameters on the load distribution at the surrounding regions. Using FEA, Chang and his collegues evaluated the pattern of micromotion within implants and surrounding bone with different thread designs (trapezoidal, buttress, square, and standard V-thread) under immediate loading of 300 N axial load.18 The results revealed that all micromotion was located near the interface of cortical and cancellous bone and the square thread profile had the most favorable micromotion value. Eraslan et al.19 performed the similar study with four different thread forms under a static axial load of 100 N. The study reported that maximum stress was concentrated at the cervical cortical regions around the first thread and the stress value was lowest in the square thread type. Likewise, other previous FEA and animal studies showed the most effective stress distribution and bone-to-implant contact area (BIC) in the square thread shape.20,21,22 Very few randomized clinical trials have evaluated the effect of thread design on osseointegration under immediate loading. However, McAllister et al.23 and Arnhart et al.24 recently performed multi-center clinical trials using variable-thread tapered implants (NobelActive, Nobel Biocare, Gothenburg, Sweden) respectively. The data from both studies demonstrated that the variable-thread tapered implants exhibited successful clinical results under demanding conditions of immediate loading. The summary of these studies is shown in Table 1.
The FEA and in vivo studies discussed above indicate that stress distribution varies with the thread shape. However, regardless of different thread designs, the maximum stress was concentrated near the cervical bone region around the first thread of the implant. In particular, the data from the FEA studies demonstrated that the square thread type had more favorable stress distribution. Using the variable-thread tapered implants, two multi-center clinical trials also showed clinical results comparable to the standard design. Although clinical evidence is limited, it is possible to conclude that the thread shape plays an important role in stress transfer between the implant and the surrounding tissue and determines the primary stability of the implant.
Thread design should maximize implant surface area and create a better spreading of stress and primary stability.25 Like thread shape, pitch is another important geometric factor that determines the bone-to-implant contact and the biomechanical load distribution. Thread pitch is defined as the distance between two neighboring threads, measured on the same side of the axis (Fig. 1).26 It also refers to the number of threads per unit length.26 Therefore, when implants have the same length, smaller pitch indicates more threads, leading to greater surface area. Another geometric parameter related to thread pitch is lead. Lead is the distance within the same thread between before and after one complete rotation in the axial direction. That is, for single-, double-, and triple-threaded implants, lead increases by one, two, and three times the pitch. Since lead indicates the distance that an implant would move after one turn, it plays an important role on determining the speed of implant insertion. Hence, thread pitch is clinically significant due to its effect on surface area and insertion speed.27
Multiple studies demonstrated that implants with smaller pitch showed the greater surface area and better stress distribution particularly in low-density bone as summarized in Table 2. Orsini et al.28 conducted an animal study placing "narrow-pitch" (0.5 mm) and "wide-pitch" (1.7 mm) implants in a sheep iliac crest model. The findings suggested that the greater BIC gained by reducing thread pitch could improve initial anchorage and primary stability in cancellous bone. Another animal study performed by Chung et al.29 also revealed that implants with a 0.6-mm pitch created more crestal bone resorption than those with a 0.5-mm pitch. In addition, the same results were found in the studies using FEA models. Authors independently reported that smaller pitch presented better load resistance and less effective stress in their three-dimensional FEA models.22,30,31 However, the optimal pitch values vary in different thread shapes. In the FEA study using triangular and trapezoidal thread forms with various pitches, Lan and his collegues recommended 1.2 mm and 1.6 mm as the optimal thread pitches of triangular- and trapezoidal-threaded implants respectively.32 For V-shape threads, the implants with a 0.8-mm pitch produced the most favorable stress distribution.33
The thread pitch determines the surface area available for load transfer to the peri-implant tissue. The common findings indicate that smaller pitch exhibits more favorable stress distribution and reinforces the primary stability of the implant. However, the optimal level of thread pitch varies in different thread design; for example, a pitch of 0.8 mm had the most effective stress distribution for V-shape threads. Lastly, thread pitch plays a more critical role in enhancing the primary stability in low-density bone than in high-density bone, so clinicians should pay close attention to this geometric factor, especially when the bone quality is poor.
In addition to thread shape and pitch, its depth and width are important design parameters that affect the stress distribution around endosteal implants. According to Misch, thread depth is the distance from the outermost tip to the innermost body of the thread (Fig. 1).34 The same author defines thread width as the distance between the superiormost and inferior-most tip of a single thread measured axially (Fig. 1).34 In other words, thread depth can also be calculated by difference between the major and minor diameter of the thread.34
Like the previously discussed geometric variables, thread depth and width clinically influence implant insertion and surface area. The shallower the thread depth, the easier the implantation procedure, especially in the highdensity bone.34 It may be able to eliminate the need for tapping during the surgery. On the contrary, deep threads increase the functional surface area at the bone-implant interface, which can improve primary stability in the low-density bone or the region with high occlusal load. Therefore, various implant systems are available in the market using progressive threads; for example, Ankylos (Dentsply Friadent, Mannheim, Germany).12 In this thread form, thread depth gradually decreases from the apical end to the coronal neck of the implant. It is allegedly claimed that it may transfer the stress away from the crestal cortical region, preventing possible bone resorption.12
Despite the advantageous effect of thread depth and width, there have been few studies designed to examine these geometric parameters. Ao's study is one of the few which evaluated the maximum von Mises stresses in the implants with various thread depths and widths under immediate loading.35 A three-dimensional FEA model was created for immediate loading applied at an axial load of 100 N and a 45-degree buccolingual load of 30 N. The thread depths and widths used in this study respectively ranged from 0.2-0.6 mm and 0.1-0.4 mm. The results revealed that thread depth affected the stress distribution more significantly than thread width. Threads with depth of more than 0.44 mm and width of 0.19-0.23 mm showed the most favorable biomechanical behavior for the immediately loaded cylinder implants designed in this study. A similar study was performed by Kong et al.36 Instead of a 45-degree buccolingual load of 30 N, 50 N was used and the rest of the FEA modeling conditions were the same as Ao's. The results were consistent with Ao's data. The optimal values of thread depth and width were 0.34-0.5 mm and 0.18-0.3 mm respectively and the thread depth was also a more sensitive factor to reduce the peak stress concentration within the bone. A more detailed description of Ao's and Kong's studies is available in Table 3.
As a result of a lack of clinical evidence, it is difficult to establish consensus guidelines for the optimal level of thread depth and width. However, similar values were obtained by both Ao and Kong's studies using the immediately loaded cylinder implants. Another common finding was that thread depth had a more significant impact on effective stress distribution than thread width. Additional in vivo studies and clinical trials are necessary to confirm the observations made in these studies.
Crest module refers to the neck portion of the implant. Implant neck configurations can be critical for minimizing the marginal bone loss. Previous studies have reported a strong association between crestal module and marginal bone resorption.37,38 This area is important for implant primary stability because the transition from an endosteal environment to an oral cavity occurs here. In addition, this is the region where the thick cortical bone is present and the occlusal stress is concentrated.27
Previously, smooth crest module was common to prevent plaque accumulation because it was exposed above the crestal plate. However, Hermann et al.39 and Hanggi et al.40 reported that shear forces increased at the crestal region when a smooth neck was inserted below the bone crest, leading to marginal bone resorption and pocket formation. Furthermore, some authors raised the concept of "disuse atrophy".41 Marginal bone resorption is caused by a smooth neck system due to its lack of exertion of the mechanical stress into the marginal bone. Therefore, Hansson introduced microthreads as the retentive elements at the neck portion to prevent the bone loss (Fig. 1).42
Studies reporting association between microthreads and marginal bone loss are listed in Table 4. Chowdhary et al.43 conducted an FEA and in vivo study to analyze the influence of microthreads on osseointegration and primary stability of implants. They placed oxidized implants with and without microthreads in the femur and tibia of rabbits. The results showed that the test group had more favorable stress concentration, bone volume and BIC than the control group. A recent animal study also compared polished and microthreaded collars and the microthreaded group exhibited less crestal bone resorption.44
In addition to the aforementioned animal study, previous clinical studies have already demonstrated reduced marginal bone loss for the microthreaded implants under conventional loading.45,46,47 Although few clinical data are available on the immediate loading in the microthreaded implants, Calvo-Guirado et al.48 recently performed a prospective study for 3 years after the implant placement under immediate loading. The implant system used in this study had microthreads up to the prosthetic platform at the neck region. The results showed 100% survival rate and minimal marginal bone loss.
Several FEA models were designed to evaluate the effect of microthread design on load transfer in the surrounding bone. The results of the two independent analyses by Amid et al.49 and Schrotenboer et al.50 indicated that addition of a microthread design increased maximum stress value at the crestal bone. This supports Wolff's law as a possible theoretical foundation for the crestal bone maintenance but still remains open to debate.
All of the studies reviewed indicate that microthreads promote bone formation and contribute to the effective stress distribution and less crestal bone resorption. Like thread pitch, microthreads plays a more critical role in preventing marginal bone loss in the cancellous bone than in the cortical bone. Therefore, the implant crestal module is an important macrodesign parameter that should be considered especially when dealing with low-density bone.
In conclusion, the biomechanical behavior of implant macrodesign factors significantly influences stress distribution at the cervical crestal bone region and ultimately primary stability under immediate loading in cancellous bone. Based on the results presented in this literature review, it was possible to draw several useful clinical conclusions about various implant macrodesign features. First, for thread shape, the square thread profile may provide the best primary stability in an immediate loading situation. When primary stability is a concern, implants with smaller pitch are beneficial by increasing BIC. Studies have shown that thread depth is more critical for dissipating peak stresses within the bone than thread width and the optimal values of thread depth and width may vary depending on thread shape and other geometric factors. Lastly, the microthread configuration at the implant neck may improve bone formation and stress distribution for the implants inserted in the cancellous bone under immediate loading.
However, information about the effect of different macrodesign characteristics should be carefully interpreted. Optimal primary stability and stress concentration are the combined results of various mechanical and biological factors. For a specific implant, the benefits from a single design feature could be enhanced or weakened by the other variables of the implant. Hence, it is important to evaluate patient's biological condition and consider various mechanical features as a whole for a particular clinical situation. Clinicians should understand that a certain design factor alone will not guarantee implant success and survival.
Few in vivo clinical studies have been conducted to investigate the influence of implant macrodesign features on primary stability of immediately loaded implants. The results of FEA and animal models may not show the same outcome in a clinical setting because they cannot completely duplicate the human clinical situation. Each individual patient has a unique biological condition such as bone quality, force distribution, masticatory activity, etc. However, these studies are relatively a simple, fast, and inexpensive approach to predict the biomechanical behavior of implants in an oral cavity compared to human studies, so they can still be an initial guide for further clinical research.
Figures and Tables
![]() | Fig. 1Implant macrodesign parameters used in this report (Implant image from Neobiotech CMI IS-II active®). |
Table 1
Studies evaluating the effect of thread shape on stress distribution
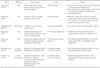
Study | Method | Thread shape | Load | Results |
---|---|---|---|---|
Chang et al. (2012)18 | FEA | Acme (trapezoidal) thread, buttress thread, sqaure thread, standard V-thread | 300 N axial load | • All micromotion was located near the interface of cortical and cancellous bone. |
• An implant with an square thread profile might provide the best primary stability under immediate loading. | ||||
Erslan et al. (2010)19 | FEA | V-thread, buttress, reverse buttress, square thread | 100 N axial load | • Maximum stress was located at cervical cortical bone regions next to the first thread. |
• The maximum von Mises stress value of the square thread type was lowest. | ||||
Steigenga et al. (2004)20 | Rabbit tibia | V-thread, reverse buttress, square thread | No intentional loading | • The square thread had significantly more BIC. |
Geng et al. (2004)21 | FEA | V-thread, thin thread, two square threads of 0.24 mm and 0.36 mm thread widths | 141 N oblique load at 45° | • V-thread and thick square thread had significantly less stress in cancellous bone. |
Chun et al. (2002)22 | FEA | Plateau type, plateau with small curvature, triangular, square, square with small radius | 100 N axial and oblique load at 15° | • The square thread with filleted with a small radius showed the most effective stress distribution. |
McAllister et al. (2012)23 | Human, 55 patients | Variable-thread tapered implant (NobelActive, Nobel Biocare) | Immediate loading, 24-month follow-up | • The variable-thread tapered implant can be a safe and effective treatment option. |
Arnhart et al. (2012)24 | Human, 177 patients | Variable-thread tapered — NAI (NobelActive internal connection), NAE (NobelActive external connection) Standard tapered - NR (NobelReplace) | Immediate loading, 36-month follow-up | • Variable-thread design showed results comparable to those of standard tapered implants. |
Table 2
Studies evaluating the effect of thread pitch on load transfer and bone-to-implant contact
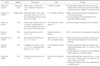
Study | Method | Thread pitch | Load | Results |
---|---|---|---|---|
Orsini et al. (2012)28 | Sheep iliac crest | "Narrow-pitch" implant (0.5 mm), "wide-pitch" implant (1.7 mm) | 8 weeks of loading | • Increasing the implant surface area by using implants with smaller pitch might be beneficial to improve primary stability in cancellous bone. |
Chung et al. (2008)29 | Beagle dogs | Branemark with 0.6 mm pitch, machined surface with 0.5 mm pitch, thermally oxidized surface with 0.5 mm pitch | 6–12 months of loading | • Mean crestal bone resorption was greatest in the Branemark group. |
• The percentage of BIC was highest in the thermally oxidized implants. | ||||
Chun et al. (2002)22 | FEA | Plateau type, plateau with small curvature, triangular with 0.7- mm pitch, square with 0.9-mm pitch, square with small radius | 100 N axial and oblique load at 15° | • Stress decreased as thread pitch decreased. |
Ma et al. (2007)30 | FEA | Implants with pitches of 0.8, 1.6, 2.4 mm | Axial and horizontal loading | • 0.8- mm pitch was more resistant to axial load. |
Motoyoshi et al. (2005)31 | FEA | Mini-implants with pitches of 0.5, 1.0, 1.5 mm | Traction force of 2 N at 45° to the bone surface | • With the abutment connected, the best stress distribution was observed in the lower pitch implants. |
Lan et al. (2012)32 | FEA | Triangular with pitches of 0.8, 1.2, 1.6 mm, trapezoidal with pitches of 1.2, 1.6 mm | 143 N axial and oblique load | • Optimal thread pitch values for triangular and trapezoidal implants were 1.2 mm and 1.6 mm respectively. |
• Thread pitch with more than 0.8 mm exhibited better results for a screwed implant. | ||||
Kong et al. (2006)33 | FEA | V-thread with pitches from 0.5 to 1.6 mm | Axial and bucco-lingual load | • Stress decreased with lower pitch from 1.6 mm up to 0.8 mm. Thread pitch with less than 0.8 mm showed more stress. |
• Thread pitch affects stress more significantly in cancellous bone. |
Table 3
Studies evaluating the effect of thread depth and width on stress distribution and bone-to-implant contact
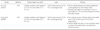
Study | Method | Thread depth and width | Load | Results |
---|---|---|---|---|
Ao et al. (2010)35 | FEA | Cylinder implants with height of 0.2–0.6 mm and width of 0.1–0.4 mm | 100 N axial load and 30 N 45° buccolingual load | • Thread depth affected the stress distribution more significantly than thread width. |
• Threads with depth of more than 0.44 mm and width of 0.19–0.23 mm showed the most favorable results. | ||||
Kong et al. (2008)36 | FEA | Cylinder implants with height of 0.2–0.6 mm and width of 0.1–0.4 mm | 100 N axial load and 50 N 45° buccolingual load | • The optimal values of thread depth and width were 0.34-0.5 mm and 0.18-0.3 mm respectively. |
• Thread depth was also a more sensitive factor to reduce the peak stress concentration within the bone. |
Table 4
Studies evaluating the effect of crestal module on marginal bone resorption
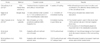
Study | Method | Crestal module | Load | Results |
---|---|---|---|---|
Chowdhary et al. (2013)43 | FEA Rabbit tibia and femur | Oxidized titanium implants with and without microthread | 4 weeks of loading | • Microthreads promote bone formation and contribute to the effective stress distribution in the cancellous bone. |
Negri et al. (2014)44 | Beagle dogs | 1.5 mm polished collar (control) vs. 0.7 mm polished and 2.5 mm microthreaded collar (test) | 1, 2, 3 months of immediate loading | • Test group showed less crestal bone resorption. |
Calvo-Guirado et al. (2014)48 | Human, 53 patients | Microthreads up to the prosthetic platform (MIS-Implants Inc) | Immediate loading, 3-year follow-up | • The implant system used in this study had 100% survival rate and minimal marginal bone loss. |
• The locations of microthreads played an important role in the stabilization process. | ||||
Amid et al. (2013)49 | FEA | Implants with and without microthreads | 100 N vertical load | • Addition of microthread design at the implant neck decreased stresses in the surrounding bone. |
Schrotenboer et al. (2008)50 | FEA | Implants with microthread or smooth neck | 100 N vertical load and 15° oblique load | • Microthreaded implants increased maximum stress value at the crestal bone. |
References
1. Haas R, Mensdorff-Pouilly N, Mailath G, Watzek G. Brånemark single tooth implants: a preliminary report of 76 implants. J Prosthet Dent. 1995; 73:274–279.
2. Goodacre CJ, Bernal G, Rungcharassaeng K, Kan JY. Clinical complications with implants and implant prostheses. J Prosthet Dent. 2003; 90:121–132.
3. Fugazzotto PA. Success and failure rates of osseointegrated implants in function in regenerated bone for 72 to 133 months. Int J Oral Maxillofac Implants. 2005; 20:77–83.
4. Atieh MA, Atieh AH, Payne AG, Duncan WJ. Immediate loading with single implant crowns: a systematic review and meta-analysis. Int J Prosthodont. 2009; 22:378–387.
5. Cochran DL, Morton D, Weber HP. Consensus statements and recommended clinical procedures regarding loading protocols for endosseous dental implants. Int J Oral Maxillofac Implants. 2004; 19:109–113.
6. Glauser R, Zembic A, Hämmerle CH. A systematic review of marginal soft tissue at implants subjected to immediate loading or immediate restoration. Clin Oral Implants Res. 2006; 17:82–92.
7. Romanos GE, Toh CG, Siar CH, Swaminathan D. Histologic and histomorphometric evaluation of peri-implant bone subjected to immediate loading: an experimental study with Macaca fascicularis. Int J Oral Maxillofac Implants. 2002; 17:44–51.
8. Ibañez JC, Jalbout ZN. Immediate loading of osseotite implants: two-year results. Implant Dent. 2002; 11:128–136.
9. Rocci A, Rocci M, Rocci C, Scoccia A, Gargari M, Martignoni M, Gottlow J, Sennerby L. Immediate loading of Brånemark system TiUnite and machined-surface implants in the posterior mandible, part II: a randomized open-ended 9-year follow-up clinical trial. Int J Oral Maxillofac Implants. 2013; 28:891–895.
10. Ostman PO, Hellman M, Sennerby L. Direct implant loading in the edentulous maxilla using a bone density-adapted surgical protocol and primary implant stability criteria for inclusion. Clin Implant Dent Relat Res. 2005; 7:S60–S69.
11. Sennerby L, Meredith N. Implant stability measurements using resonance frequency analysis: biological and biomechanical aspects and clinical implications. Periodontol 2000. 2008; 47:51–66.
12. Abuhussein H, Pagni G, Rebaudi A, Wang HL. The effect of thread pattern upon implant osseointegration. Clin Oral Implants Res. 2010; 21:129–136.
13. Boggan RS, Strong JT, Misch CE, Bidez MW. Influence of hex geometry and prosthetic table width on static and fatigue strength of dental implants. J Prosthet Dent. 1999; 82:436–440.
14. Hansson S, Werke M. The implant thread as a retention element in cortical bone: the effect of thread size and thread profile: a finite element study. J Biomech. 2003; 36:1247–1258.
15. Prendergast PJ, Huiskes R. Microdamage and osteocyte-lacuna strain in bone: a microstructural finite element analysis. J Biomech Eng. 1996; 118:240–246.
16. Brunski JB. In vivo bone response to biomechanical loading at the bone/dental-implant interface. Adv Dent Res. 1999; 13:99–119.
17. Frost HM. Skeletal structural adaptations to mechanical usage (SATMU): 1. Redefining Wolff's law: the bone modeling problem. Anat Rec. 1990; 226:403–413.
18. Chang PK, Chen YC, Huang CC, Lu WH, Chen YC, Tsai HH. Distribution of micromotion in implants and alveolar bone with different thread profiles in immediate loading: a finite element study. Int J Oral Maxillofac Implants. 2012; 27:e96–e101.
19. Eraslan O, Inan O. The effect of thread design on stress distribution in a solid screw implant: a 3D finite element analysis. Clin Oral Investig. 2010; 14:411–416.
20. Steigenga J, Al-Shammari K, Misch C, Nociti FH Jr, Wang HL. Effects of implant thread geometry on percentage of osseointegration and resistance to reverse torque in the tibia of rabbits. J Periodontol. 2004; 75:1233–1241.
21. Geng JP, Ma QS, Xu W, Tan KB, Liu GR. Finite element analysis of four thread-form configurations in a stepped screw implant. J Oral Rehabil. 2004; 31:233–239.
22. Chun HJ, Cheong SY, Han JH, Heo SJ, Chung JP, Rhyu IC, Choi YC, Baik HK, Ku Y, Kim MH. Evaluation of design parameters of osseointegrated dental implants using finite element analysis. J Oral Rehabil. 2002; 29:565–574.
23. McAllister BS, Cherry JE, Kolinski ML, Parrish KD, Pumphrey DW, Schroering RL. Two-year evaluation of a variable-thread tapered implant in extraction sites with immediate temporization: a multicenter clinical trial. Int J Oral Maxillofac Implants. 2012; 27:611–618.
24. Arnhart C, Kielbassa AM, Martinez-de Fuentes R, Goldstein M, Jackowski J, Lorenzoni M, Maiorana C, Mericske-Stern R, Pozzi A, Rompen E, Sanz M, Strub JR. Comparison of variable-thread tapered implant designs to a standard tapered implant design after immediate loading. A 3-year multicentre randomised controlled trial. Eur J Oral Implantol. 2012; 5:123–136.
25. Ivanoff CJ, Gröndahl K, Sennerby L, Bergström C, Lekholm U. Influence of variations in implant diameters: a 3- to 5-year retrospective clinical report. Int J Oral Maxillofac Implants. 1999; 14:173–180.
26. Misch CE, Steignga J, Barboza E, Misch-Dietsh F, Cianciola LJ, Kazor C. Short dental implants in posterior partial edentulism: a multicenter retrospective 6-year case series study. J Periodontol. 2006; 77:1340–1347.
27. Steigenga JT, al-Shammari KF, Nociti FH, Misch CE, Wang HL. Dental implant design and its relationship to long-term implant success. Implant Dent. 2003; 12:306–317.
28. Orsini E, Giavaresi G, Trirè A, Ottani V, Salgarello S. Dental implant thread pitch and its influence on the osseointegration process: an in vivo comparison study. Int J Oral Maxillofac Implants. 2012; 27:383–392.
29. Chung SH, Heo SJ, Koak JY, Kim SK, Lee JB, Han JS, Han CH, Rhyu IC, Lee SJ. Effects of implant geometry and surface treatment on osseointegration after functional loading: a dog study. J Oral Rehabil. 2008; 35:229–236.
30. Ma P, Liu HC, Li DH, Lin S, Shi Z, Peng QJ. Influence of helix angle and density on primary stability of immediately loaded dental implants: three-dimensional finite element analysis. Zhonghua Kou Qiang Yi Xue Za Zhi. 2007; 42:618–621.
31. Motoyoshi M, Yano S, Tsuruoka T, Shimizu N. Biomechanical effect of abutment on stability of orthodontic mini-implant. A finite element analysis. Clin Oral Implants Res. 2005; 16:480–485.
32. Lan TH, Du JK, Pan CY, Lee HE, Chung WH. Biomechanical analysis of alveolar bone stress around implants with different thread designs and pitches in the mandibular molar area. Clin Oral Investig. 2012; 16:363–369.
33. Kong L, Liu BL, Hu KJ, Li DH, Song YL, Ma P, Yang J. Optimized thread pitch design and stress analysis of the cylinder screwed dental implant. Hua Xi Kou Qiang Yi Xue Za Zhi. 2006; 24:509–512. 515
34. Misch CE, Strong T, Bidez MW. Scientific rationale for dental implant design. In : Misch CE, editor. Contemporary Implant Dentistry. 3rd ed. St. Louis: Mosby;2008. p. 200–229.
35. Ao J, Li T, Liu Y, Ding Y, Wu G, Hu K, Kong L. Optimal design of thread height and width on an immediately loaded cylinder implant: a finite element analysis. Comput Biol Med. 2010; 40:681–686.
36. Kong L, Hu K, Li D, Song Y, Yang J, Wu Z, Liu B. Evaluation of the cylinder implant thread height and width: a 3-dimensional finite element analysis. Int J Oral Maxillofac Implants. 2008; 23:65–74.
37. Quirynen M, Naert I, van Steenberghe D. Fixture design and overload influence marginal bone loss and fixture success in the Brånemark system. Clin Oral Implants Res. 1992; 3:104–111.
38. Shimada E, Pilliar RM, Deporter DA, Schroering R, Atenafu E. A pilot study to assess the performance of a partially threaded sintered porous-surfaced dental implant in the dog mandible. Int J Oral Maxillofac Implants. 2007; 22:948–954.
39. Hermann JS, Schoolfield JD, Nummikoski PV, Buser D, Schenk RK, Cochran DL. Crestal bone changes around titanium implants: a methodologic study comparing linear radiographic with histometric measurements. Int J Oral Maxillofac Implants. 2001; 16:475–485.
40. Hänggi MP, Hänggi DC, Schoolfield JD, Meyer J, Cochran DL, Hermann JS. Crestal bone changes around titanium implants. Part I: A retrospective radiographic evaluation in humans comparing two non-submerged implant designs with different machined collar lengths. J Periodontol. 2005; 76:791–802.
41. Vaillancourt H, Pilliar RM, McCammond D. Finite element analysis of crestal bone loss around porous-coated dental implants. J Appl Biomater. 1995; 6:267–282.
42. Hansson S. The implant neck: smooth or provided with retention elements. A biomechanical approach. Clin Oral Implants Res. 1999; 10:394–405.
43. Chowdhary R, Halldin A, Jimbo R, Wennerberg A. Influence of micro threads alteration on osseointegration and primary stability of implants: an FEA and in vivo analysis in rabbits. Clin Implant Dent Relat Res. 2013; 08. 27.
44. Negri B, Calvo Guirado JL, Maté Sánchez de Val JE, Delgado >Ruíz RA, Ramírez Fernández MP, Barona Dorado C. Peri-implant tissue reactions to immediate nonocclusal loaded implants with different collar design: an experimental study in dogs. Clin Oral Implants Res. 2014; 25:e54–e63.
45. Yun HJ, Park JC, Yun JH, Jung UW, Kim CS, Choi SH, Cho KS. A short-term clinical study of marginal bone level change around microthreaded and platform-switched implants. J Periodontal Implant Sci. 2011; 41:211–217.
46. Song DW, Lee DW, Kim CK, Park KH, Moon IS. Comparative analysis of peri-implant marginal bone loss based on microthread location: a 1-year prospective study after loading. J Periodontol. 2009; 80:1937–1944.
47. Lee DW, Choi YS, Park KH, Kim CS, Moon IS. Effect of microthread on the maintenance of marginal bone level: a 3-year prospective study. Clin Oral Implants Res. 2007; 18:465–470.
48. Calvo-Guirado JL, Gómez-Moreno G, Aguilar-Salvatierra A, Guardia J, Delgado-Ruiz RA, Romanos GE. Marginal bone loss evaluation around immediate non-occlusal microthreaded implants placed in fresh extraction sockets in the maxilla: a 3-year study. Clin Oral Implants Res. 2014; 01. 15.
49. Amid R, Raoofi S, Kadkhodazadeh M, Movahhedi MR, Khademi M. Effect of microthread design of dental implants on stress and strain patterns: a three-dimensional finite element analysis. Biomed Tech (Berl). 2013; 58:457–467.
50. Schrotenboer J, Tsao YP, Kinariwala V, Wang HL. Effect of microthreads and platform switching on crestal bone stress levels: a finite element analysis. J Periodontol. 2008; 79:2166–2172.