Abstract
This study was conducted to assess the feasibility of image guided radiotherapy (IGRT) for orthotopic 4T1 mouse mammary tumor using linear accelerator (LINAC). Eighteen Balb/C mice were inoculated with 4T1 cells on left mammary fat pad and nine of them were irradiated using LINAC. Tumors, planning target volumes (PTV), bowels adjacent to tumors, bones and lungs were delineated on planning CT images. IGRT plans were generated to irradiate prescription dose to at least 90% of the PTV and then compared with conventional 2-dimensional plans with anterior-posterior and posterior-anterior beams with 5 mm margins (2D AP/PA plan). Homemade dose-build-up-cradle was designed to encompass mouse bed for homogeneous dose build up. To confirm the irradiated dose, tumor doses were measured using diode detector placed on the surface of tumors. Plan comparison demonstrated equivalent doses to PTV while sparing more doses to normal tissues including bowel (from 90.9% to 40.5%, median value of mean doses) and bone marrow (from 12.9% to 4.7%, median value of mean doses) than 2D AP/PA plan. Quality assurance using diode detector confirmed that IGRT could deliver 95.3-105.3% of the planned doses to PTV. Tumors grew 505.2-1185.8% (mean 873.3%) in the control group and 436.1-771.8% (mean 615.5%) in the irradiated group. These results demonstrate that LINAC-based IGRT provides a reliable approach with accurate dose delivery in the radiobiological study for orthotropic tumor model maintaining tumor microenvironment.
Preclinical experiments using cancer cell lines or animal models have been profoundly conducted in order to study the effect of chemotherapeutic agents, target agents and radiation. The experiments using the cell line have some advantages as compared with the animal models; these include relatively short study period and easy interpretation of the results. However, living body is not composed of single-type cells. Various types of cells are arranged in an orderly fashion to form a living creature and they are controlled by the effect of cell-to-cell contact, cytokines, hormones and immune system. Not only the inherent characteristics of the cancer cells but also these interactions and tumor vascularity are mainly involved in the development, growth and death of tumor. Treatment response and development of resistance for certain treatment modality in certain tumor are also influenced by these systemic effects of the whole body. While cell line-based experiment reflects limited effect of chemotherapy or radiation therapy, animal tumor model study can complement a large part of its disadvantages. Moreover, orthotopic tumor model, where tumor cells are inoculated and grown in the original tumor location, is considered more clinically relevant and better predictive for the treatment efficacy as compared with standard subcutaneous models [1,2]. These tumors reflect the original situation, including various microenvironments.
For animal experiments, linear accelerator (LINAC) used in clinic or specially designed equipments are used, but the importance of technique of radiation delivery or dose verification is not considered seriously and usually not fully described. Beam shaping and shielding of normal tissue is not easy for small field irradiation, i.e., the width <3 cm. Accordingly, in many studies, tumors are usually implanted in the subcutaneous tissue of thigh or flank with no respect to the type of cancer and they are irradiated at a margin of 5 mm [3,4,5,6]. In the head-and-neck tumor study, the whole head is irradiated [7]. In a murine model of orthotopic breast cancer, however, radiosensitive organs, such as bowel, liver and bladder, are located proximal to the mammary fat pad. Furthermore, when researchers attempt to examine the systemic effects of focal ablative radiation to the target tumor, they should pay special attention. They should limit the radiation doses given to normal tissues such as bone marrow, spleen, lymph nodes or other immune system organs that are involved in the host immunity.
Image-guided radiation therapy (IGRT) is a procedure that refines the delivery of therapeutic radiation by applying image-based target re-localization and proper re-positioning to achieve accurate target coverage, thus minimizing the volume of normal tissue exposed to ionizing radiation [8]. With the recent technological advances in the radiation therapy, latest LINACs equipped with cone beam CT (CBCT) can conduct IGRT for the purposes of checking the treated area with millimeter-scale accuracy. Moreover, it is also possible not only to shield organs at risk (OARs) and other normal tissue precisely using a 2.5-mm width multileaf collimator (MLC) but also to obtain a sharp dose gradient focusing a high-dose radiation on the tumor, as shown in radiosurgery or stereotactic body radiotherapy. If the animal experiment is conducted using this type of LINAC, the consistency of radiation dose delivery may be well maintained while preserving not only the organ function of irradiated site but also other normal functions, including hematopoietic and immune system. This may broaden the scope of radiobiological study of animal tumor model.
In this study, we examined the feasibility of LINAC based IGRT for orthotopic 4T1 mouse mammary tumor, thus attempting to establish radiobiological animal study method for maintaining tumor microenvironments.
Eighteen, five-week-old healthy immuno-competent BALB/c mice with normal physiological functions were obtained from Orient Bio. All mice were maintained under specific pathogen free conditions and used in accordance with the Institutional Animal Care and Use Committee Guidelines of Gachon University. When mice were 7-week-old (day 0), mice were orthotopically injected with 4T1 (5×104/µL PBS) cells in the left fourth mammary fat pad. Tumor sizes were measured at a 2 or 3-day interval using electronic calipers and tumor volumes were calculated as length×width2×0.52. The weight of mice was also measured and recorded with tumor size. On day 14, mice were divided into two groups, comprising nine mice each. Thus, we ensured that tumor sizes and body weights of the mice were evenly distributed.
To ensure the surface dose build-up of 6 MV photon beam for the superficially located murine orthotopic tumor, grown in the mammary fat pad just beneath the skin, homemade dose-build-up cradle was designed. Three acrylic plates of size of 30.0 cm×30.0 cm×0.6 cm were attached together to make 1.8 cm thickness on the anterior direction of the cradle. U-shaped 5 cm thickness pillar, made of Styrofoam, encompassed a mouse bed inside. On the posterior direction, two acrylic plates of the same size were attached considering the thickness of mouse bed (Figure 1a). The mouse bed was made of acrylic plate of 14.0 cm×18.0 cm×0.5 cm and placed inside the cradle. Stand bar was attached in the head direction of the bed to make slope so that a mouse was naturally extended when the upper incisors of mouse were hung on threads at the top of the bed (Figure 1b).
To investigate the efficacy of dose-build-up cradle for building up of surface radiation dose, it was placed on the treatment couch of LINAC with the mouse bed inside. Tissue-equivalent bolus of 2 cm thickness was laid on the bed, taking into account the thickness of mouse, which is about 2 cm or less. The doses of surface, center and bottom of bolus were measured with diode detector (Figure 2). To check whether margin of 5 mm commonly used in animal experiment is appropriate, portal vision image was obtained using electronic portal imaging device (EPID) during irradiation and density profile perpendicular to the border of treatment field was analyzed.
All mice underwent CT simulation to obtain radiation therapy planning CT images. They were anesthetized by intraperitoneal injection of ketamine (100 mg/kg) and xylazine (10 mg/kg) and immobilized on the top of the mouse bed hanging upper incisors up on the threads. CT images of 1 mm slice thickness were obtained and then transferred to RT planning software, Eclipse version 10.0 (Varian, Palo Alto, USA). Tumors, bowels which are adjacent to tumor, bone, bladder, and lungs were contoured on each CT images. Planning target volumes
were generated from tumor with a 1-mm margin.
To validate the efficacy of the IGRT planning and treatment, two kinds of planning were performed and compared. First, two-dimensional plans with opposing fields were done (2D AP/PA plan), in which tumor was irradiated using anterior-posterior and posterior-anterior beams with 5 mm margin (Figure 3). Second, image guided radiation plans were generated using two fields at various gantry angles (IGRT plan, Figure 4) and compared with 2D AP/PA plan. Taking into account the location of tumor implanted on mammary fat pad, beam arrangements were devised to minimize doses to OARs and 2.5 mm width MLCs were used to shield normal tissue while maintaining PTV plus 5 mm plan margin. The planning objective was to deliver the prescription dose to more than 90% of the PTV. The dose volume histograms of the 2 plan strategies were obtained and compared for doses to PTV, bowels adjacent to tumor, bone and lungs.
All mice were treated using Novalis Tx™ (Varian, Palo Alto, USA) equipped with a 2.5-mm width MLC. Irradiation was delivered using a 6-MV photon beam at a dose rate of 6 00 monitor unit/min. Total 12 Gy in 3 fractions was given at 24-hour intervals. Before each treatment, mice were anesthetized and immobilized on the mouse bed in a dose-build-up cradle in the same manner as the CT simulation. Then, cone beam CT images were obtained and fused with planning CT images. Treatment couch of Novalis Tx™ was adjusted accordingly as the coordinate information derived from the matching of the CBCT and planning CT data. After the adjustment of the treatment couch, electronic portal images were obtained to confirm target positions just before treatment. During the treatment, actual doses were measured using diode detector located on the surface of the tumors in all mice to ensure that planned doses were delivered (Figure 5).
Tumor growth and body weight were evaluated at a 2 or 3-day interval until death or sacrifice of the mice. Tumor volumes were calculated as length×width2×0.52 using electronic calipers. Sacrifices were done according to the institutional guidelines; when tumor diameter exceeded 2 cm, tumor weights exceeded 10% of body weight, or mice showed dyspnea, abnormal posture, >20% body weight loss, or any other clinical sign of metastatic disease causing significant pain or distress. On day 28, tumors and lungs were harvested and tumor volume, tumor weight and number of metastatic nodules on the surface of lungs were measured.
Assuming that the measured value at the center of the bolus on mouse bed placed in a dose-build-up cradle was 100, measured values at the surface and bottom were both 97.7. Since the dose distribution from surface to bottom was consistent (dose variation of 2.4%), tumor dose of the mouse could be measured by surface dose using a dose-build-up cradle. Tumor doses measured on the surface of the tumor using diode were 95.3-105.3% of the calculated dose in RT plans.
As compared with 2D AP/PA plan, IGRT plan could deliver equivalent dose to PTV while sparing normal tissues (Figure 6). When the prescription dose was irradiated to more than 90% of PTV, mean PTV doses were 103.1-107.7% (median 104.1%) in IGRT plan and 102.4-103.3% (median 102.8%), respectively. Maximum and mean doses to bowels adjacent to tumor and mean dose to bone were significantly lower in IGRT plan (Table 1). Lungs were 8.2-10.6 mm (median 9.6 mm) away from the treatment field edge and lung doses were negligible in both groups; lung volumes irradiated more than 1% of prescription dose were less than 1.5% in both groups.
Density profile of electronic portal image, perpendicular to the border of treatment field of 2.5 cm×2.0 cm, showed that rapid dose fall off occurs within ±2 mm from the treatment field edge (Figure 7). It took less than 10 minutes from mice set-up to the end of the treatment.
All mice in the treatment group survived until the end of the experiment. RT-related toxicities such as skin reaction, changes in eating habits, or diarrhea were not observed. The mean body weights of mice decreased 1.1% in control group and 2.7% in irradiated group, respectively (Table 2). The mean body weights at necropsy (day 28) were 18.4 g in control group and 18.1 g in treatment group, respectively, and there was no significant difference between both groups (P=0.273). No gross bowel change was found at necropsy. The relative tumor growths from baseline in day 14 were 505.2-1185.8% (mean 873.3%) in the control group and 436.1-771.8% (mean 615.5%) in the RT group, respectively (P=0.023). There were no significant differences in the numbers of metastatic nodules on the surface of lungs between the two groups (Figure 8).
The tumor microenvironment is the cellular and subcellular environment in which the tumor cell exists, including the extracellular matrix, fibroblasts, surrounding blood vessels, immune cells, cytokines, hormones and other signaling molecules. Hanahan et al. reported that "the biology of tumors can no longer be understood simply by enumerating the traits of the cancer cells but instead must encompass the contributions of the tumor microenvironment to tumorigenesis" [9]. However, many experiments with radiation have used cell lines and these cell line-based studies cannot reflect the influence of the tumor microenvironment at all. In some research using animals, tumor microenvironment should be maintained as similar to the actual treatment as possible. For technical convenience, however, tumor cells have been inoculated into the subcutaneous tissue of thigh or flank in many murine tumor model studies. If the tumor cells are inoculated on flank and grow up at the end of the body, it is easy to deliver desired radiation dose with little concerns of radiation exposure of normal tissue. For example, AP 1-field or AP/PA 2-fields technique can simply reduce the irradiated area of the abdomen with the simple shield. Thigh is relatively away from the organs at risk, such as bowel, spinal cord or kidney, so that obliquely positioned mice can reduce irradiated dose to normal tissue and 4 or 5 mice can be treated simultaneously (Figure 9). However, as the subcutaneous tissue of the thigh or flank is not the site of the original tumor and merely serves as the bed that tumor cells can survive, these models also cannot fully represent the tumor microenvironment.
4T1 mouse mammary cancer cell line has rapid growth pattern and high metastatic potential to lung and it is frequently used for the study of highly aggressive breast cancer biology. If 4T1 cells are inoculated in mammary fat pad, the tumor specific microenvironment is well preserved and lung metastasis occurs frequently so that this model is suitable for appropriate analysis of the therapeutic efficacy of certain treatment modality. For the radiobiological study using 4T1 cells, the major obstacle is the proximity of mouse mammary fat pad to bowel, bladder, spine and pelvic bone when they are orthotopically inoculated. When conventional RT with simple shield technique with single AP field beam is used, large portion of abdomen may be irradiated so that a high dose radiation treatment cannot be performed. To overcome this problem, tumor location-specific RT plan with LINAC based IGRT using CBCT was tested in the present study. The authors suggest that LINAC based IGRT is effective for tumor control while irradiating lower dose to abdomen and maintaining tumor microenvironment. Although it took longer than simultaneous treatment, IGRT was possible to be carried out within 10 minutes per mouse.
In animal study using radiation, dose verification is as much important as location of the tumor. If it is not verified that prescribed dose was actually irradiated, the relationship between treatment efficacy and radiation dose cannot be trusted. Since the kilovoltage irradiators, mainly used in a cell line-based experiment, have relatively large heel effect, dose distribution becomes non-uniform in the irradiated field, which means some cells may irradiated more or less. To compensate this phenomenon, mounted treatment table should be rotated during irradiation. Suggested dose verification method for this rotating irradiator table is film dosimetry. It is easy to setup with reliable measurement results.
On the other hand, LINAC produces electrons in the megavoltage range and the x-ray intensity is peaked in the forward direction so that the heel effect is negligible [10]. In addition, to make the beam intensity uniform across the field, a flattening filter is inserted in the midst of beam tract. Therefore, dose uniformity is reliable within the treatment field in LINAC with symmetry variation of <2%. Because the thickness of mouse body is usually less than 2 cm, dose build up and selection of the detector are essential rather than dose uniformity in mouse study using megavoltage beam. We manufactured dose-build-up cradle to build up tumor surface dose because tumors were located just beneath the skin. Three acrylic plates to anterior direction and two plates to posterior direction were used as a beam spoiler and satisfactory even dose distributions were obtained. Several authors placed bolus on the surface of the tumor but the bolus frequently adheres to mouse hair. In addition, the body shape of mice or location of tumor is changed because of the relatively high weight of the bolus itself.
In large fields, air-field ionization chambers are usually the first choice but different types of detectors are in use in small field dosimetry [11]. Since the field size was 2.5 cm×2.0 cm in the current study, pinpoint chambers, silicon diodes, diamond detectors, or plastic scintillation detectors could be used. We measured the actual dose using diode detector because only diode was possible to be placed on the surface of the tumor. The variation of measured dose on surface, center and bottom of the bolus of 2 cm thickness in the dose-build-up cradle was less than 2.4%. Consequently, actual tumor surface doses using a diode were 95.3-105.3% of the calculated dose in RT plans and we could successfully verify that prescribed dose was actually well irradiated to tumor. Considering the inherent uncertainty of diode (±3%), positioning error and small field size [12], this dose variation is within acceptable range.
Margin of 5 mm has been reported from many animal experiments using mice [3,4,5,7]. The definition of geometric field border is 50% of the dose at the central axis in a plane perpendicular to the central axis of the beam [13]. Density profile around the border of the geometric field showed that rapid dose fall off occurs within ±2 mm from the treatment field edge. It is therefore considered that margin of 5 mm is sufficient for small field size in mouse model study and the outer area which is 2-3 mm from the field edge is shielded well (Figure 7).
In the treatment of human cancer, LINAC-based radiosurgery or stereotactic body radiotherapy has been widely implemented with ablative high dose radiation, usually 16 to 24 Gy in single fraction or 8 Gy or more per fraction in fractionated treatments. The clinical results demonstrated high local tumor control [14,15]. Although several data suggest that vascular damage and immune stimulation play an important role [16,17], exact biologic mechanisms of the improved tumor control rate has not been fully understood. The orthotopic animal tumor studies which maintain the tumor microenvironments with high dose irradiation using more than 10 Gy per fraction, would provide a clue to uncover these mechanisms. It is commonly difficult to give high dose radiation with conventional irradiation technique because organs at risk are close to the tumor in orthotopic tumor system. In addition, if a large portion of bones are irradiated, bone marrow cells are destroyed and immune system is deteriorated. From this context, IGRT will be a good methodology. The LINAC-based IGRT in the present study demonstrated its feasibility to deliver prescribed dose to the tumor target with easy. IGRT can be used to give high radiation dose with millimeter accuracy as used in radiosurgery or stereotactic body radiotherapy. We have plans to conduct stereotactic body radiotherapy with prescription doses of 10 Gy or more per fraction in orthotopic mouse 4T1 mammary tumor. It might give some clue to understand radiobiological mechanism of ablative dose radiation including possible systemic immune reaction.
In summary, it is feasible to treat orthotopic 4T1 mouse mammary tumor with LINAC-based IGRT. Because of megavoltage LINAC beam, dose build-up is essential for increasing the surface dose of tumor grown in a small-sized animal model. Homemade dose-build-up cradle was successfully helpful for making acceptable even dose distributions within the tumor target. Accurate dose delivery to targets limiting more doses to normal tissue including bowel and bone marrow than 2D AP/PA plan was demonstrated. Tumor growth delay assay confirmed its radiation effect. IGRT provides a reliable approach to radiobiological study for small animal orthotropic tumor model maintaining tumor microenvironment.
Acknowledgments
This work was supported by Gachon University Gil Medical Center. (Grant number: 2013-42)
References
1. Fidler IJ, Wilmanns C, Staroselsky A, Radinsky R, Dong Z, Fan D. Modulation of tumor cell response to chemotherapy by the organ environment. Cancer Metastasis Rev. 1994; 13(2):209–222. PMID: 7923551.


2. Kuo TH, Kubota T, Watanabe M, Furukawa T, Kase S, Tanino H, Saikawa Y, Ishibiki K, Kitajima M, Hoffman RM. Site-specific chemosensitivity of human small-cell lung carcinoma growing orthotopically compared to subcutaneously in SCID mice: the importance of orthotopic models to obtain relevant drug evaluation data. Anticancer Res. 1993; 13(3):627–630. PMID: 8391244.
3. Demaria S, Kawashima N, Yang AM, Devitt ML, Babb JS, Allison JP, Formenti SC. Immune-mediated inhibition of metastases after treatment with local radiation and CTLA-4 blockade in a mouse model of breast cancer. Clin Cancer Res. 2005; 11(2 Pt 1):728–734. PMID: 15701862.
4. Pilones KA, Kawashima N, Yang AM, Babb JS, Formenti SC, Demaria S. Invariant natural killer T cells regulate breast cancer response to radiation and CTLA-4 blockade. Clin Cancer Res. 2009; 15(2):597–606. PMID: 19147765.


5. Bouquet F, Pal A, Pilones KA, Demaria S, Hann B, Akhurst RJ, Babb JS, Lonning SM, DeWyngaert JK, Formenti SC, Barcellos-Hoff MH. TGFâ1 inhibition increases the radiosensitivity of breast cancer cells in vitro and promotes tumor control by radiation in vivo. Clin Cancer Res. 2011; 17(21):6754–6765. PMID: 22028490.


6. Verbrugge I, Hagekyriakou J, Sharp LL, Galli M, West A, McLaughlin NM, Duret H, Yagita H, Johnstone RW, Smyth MJ, Haynes NM. Radiotherapy increases the permissiveness of established mammary tumors to rejection by immunomodulatory antibodies. Cancer Res. 2012; 72(13):3163–3174. PMID: 22570253.


7. Wang WC, Liang SL, Chen YK, Lin LM. The therapeutic effect of fractionated radiation on DMBA-induced hamster buccal pouch squamous cell carcinomas. Oral Oncol. 2008; 44(12):1160–1166. PMID: 18486531.


8. Potters L, Gaspar LE, Kavanagh B, Galvin JM, Hartford AC, Hevezi JM, Kupelian PA, Mohiden N, Samuels MA, Timmerman R, Tripuraneni P, Vlachaki MT, Xing L, Rosenthal SA. American Society for Therapeutic Radiology and Oncology (ASTRO) and American College of Radiology (ACR) practice guidelines for image-guided radiation therapy (IGRT). Int J Radiat Oncol Biol Phys. 2010; 76(2):319–325. PMID: 20117284.


9. Hanahan D, Weinberg RA. Hallmarks of cancer: the next generation. Cell. 2011; 144(5):646–674. PMID: 21376230.


10. Khan F. Clinical radiation generators. The physics of radiation therapy. 4th ed. Lippincott Williams & Wilkins;2009. p. 35–53.
11. Wuerfel J. Dose measurements in small fields. Med Phys Int J. 2013; 1(1):81–90.
12. Saini AS, Zhu TC. Energy dependence of commercially available diode detectors for in-vivo dosimetry. Med Phys. 2007; 34(5):1704–1711. PMID: 17555252.
13. Khan F. Treatment Planning I: Isodose Distributions. The physics of radiation therapy. 4th ed. Lippincott Williams & Wilkins;2009. p. 176–200.
14. Balagamwala EH, Angelov L, Koyfman SA, Suh JH, Reddy CA, Djemil T, Hunter GK, Xia P, Chao ST. Single-fraction stereotactic body radiotherapy for spinal metastases from renal cell carcinoma. J Neurosurg Spine. 2012; 17(6):556–564. PMID: 23020208.


15. Stinauer MA, Kavanagh BD, Schefter TE, Gonzalez R, Flaig T, Lewis K, Robinson W, Chidel M, Glode M, Raben D. Stereotactic body radiation therapy for melanoma and renal cell carcinoma: impact of single fraction equivalent dose on local control. Radiat Oncol. 2011; 6:34. PMID: 21477295.


16. Song CW, Cho LC, Yuan J, Dusenbery KE, Griffin RJ, Levitt SH. Radiobiology of stereotactic body radiation therapy/stereotactic radiosurgery and the linear-quadratic model. Int J Radiat Oncol Biol Phys. 2013; 87(1):18–19. PMID: 23608235.


17. Maehata Y, Onishi H, Kuriyama K, Aoki S, Araya M, Saito R, Tominaga L, Oguri M, Araki T. Immune responses following stereotactic body radiotherapy for stage I primary lung cancer. Biomed Res Int. 2013; 2013:731346. PMID: 24324972.


Figure 1
Mouse bed and dose-build-up cradle made of acrylic plate. (A) Three acrylic plates of 30 cm×30 cm×0.6 cm on anterior direction and two on posterior direction were attached together. U-shaped pillar was made of Styrofoam. (B) When a mouse is laid on mouse bed, the body of the mouse is naturally extended because of the slope.
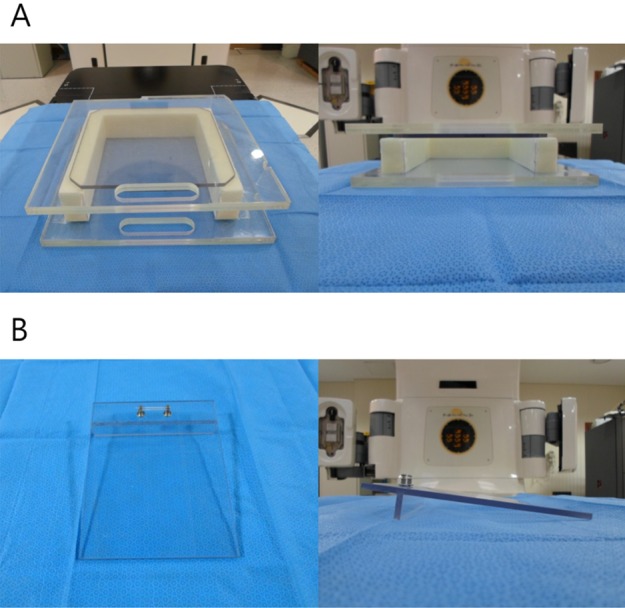
Figure 2
(A) Two tissue-equivalent boluses of 2 cm-thickness located on the mouse bed in dose-build-up cradle. (B) Dose measurement points of bolus. The numbers of 1, 2 and 3 indicate the surface, center and bottom of bolus, respectively.
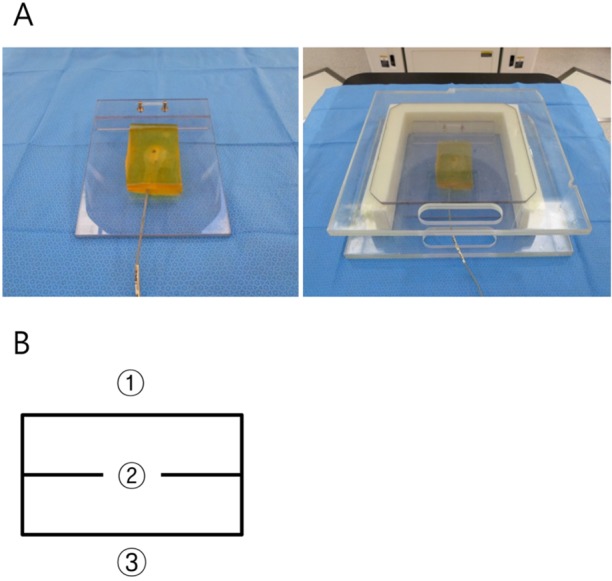
Figure 3
Axial, sagittal and coronal view of 2 dimensional anterior-posterior/posterior-anterior plan. Field margin was 5 mm from planning target volume. It is shown that a part of bowel adjacent to tumor is included within the treatment field.
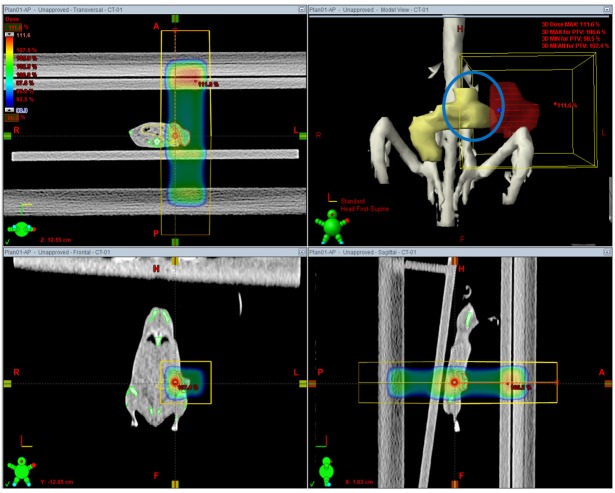
Figure 4
Axial, sagittal and coronal view of image guided radiation therapy plan. Field margin was 5 mm from planning target volume. The volume of irradiated bowel is reduced because of adjustment of beam direction and appropriate beam shaping with multileaf collimators.
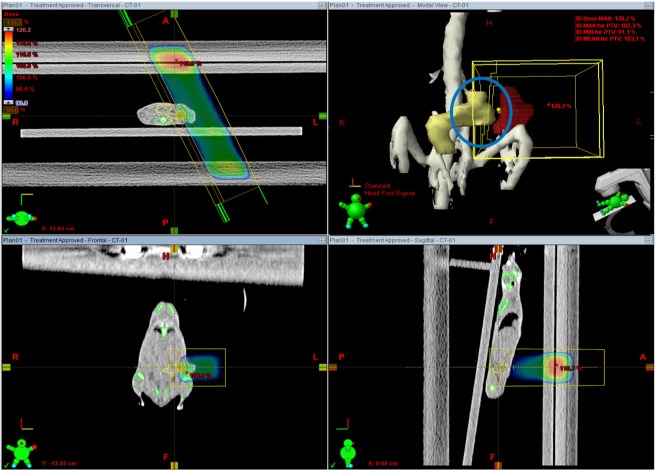
Figure 5
(A) Cone beam CT images were fused with digitally reconstructed radiogram generated from planning CT images to confirm the tumor location. (B) Open-field portal vision image obtained by electronic portal imaging device just before the treatment, which shows well matched tumor location. (C) In vivo tumor dosimetry using diode detector placed on the tumor surface.
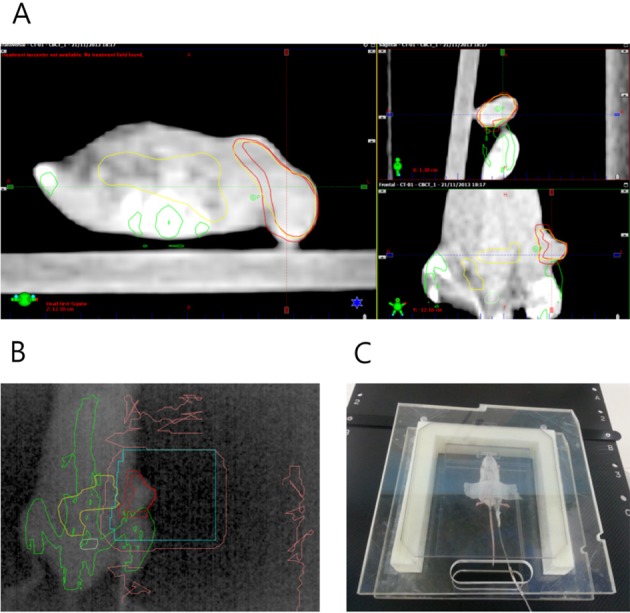
Figure 6
An example of dose volume histogram of 2-dimensional anterior-posterior/posterior-anterior (2D AP/PA) field plan and image guided radiation therapy (IGRT) plan. In IGRT plan, tumor dose is higher and that of the bowel, bone and bladder is lower as compared with 2D AP/PA field plan.
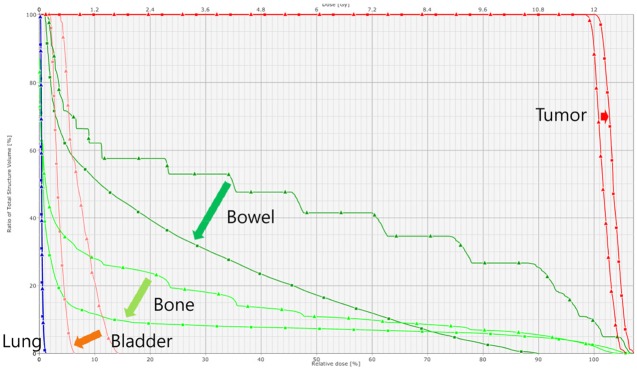
Figure 7
An example of density profile of the treatment field using electronic portal image. Rapid dose fall off occurs within ±2 mm from the treatment field edge.
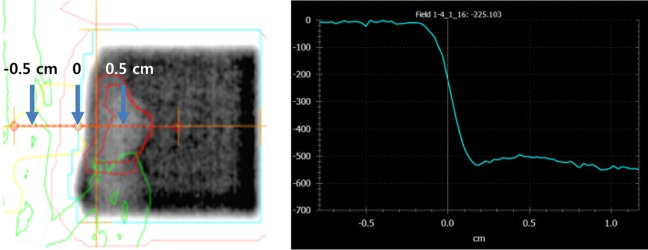
Figure 8
Tumor growth delay and metastatic lung nodules. (A) Primary tumor growth measured by electronic calipers in vivo. (B) Primary tumor volume after necropsy on day 28. (C) Metastatic lung nodules observed at necropsy on day 28.
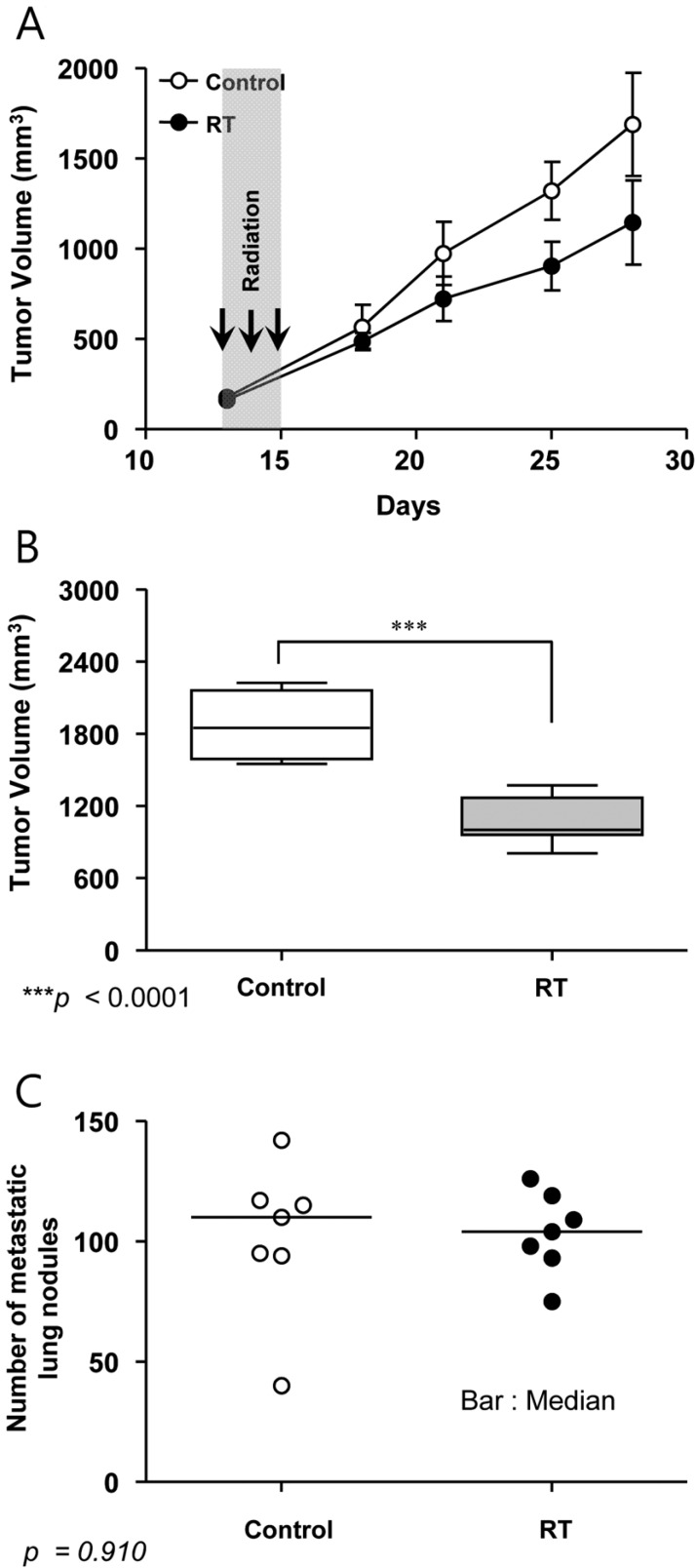
Figure 9
Simultaneous treatment of tumors inoculated on the thigh. Mice were obliquely positioned to reduce the irradiated dose to normal tissue of abdomen. Five mice were treated simultaneously.
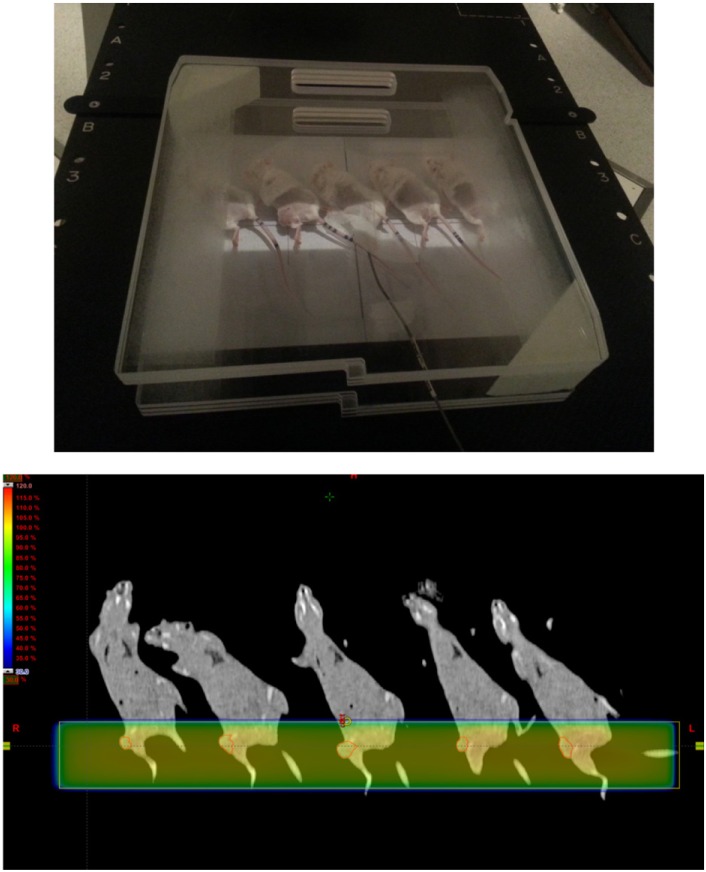