This article has been corrected. See "Erratum: Bone regeneration of mouse critical-sized calvarial defects with human mesenchymal stem cells in scaffold" in Volume 30 on page 44.
Abstract
Combination of tissue engineering and cell therapy represents a promising approach for bone regeneration. Human mesenchymal stem cells (hMSCs) have properties that include low immunogenicity, high proliferation rate, and multi-differentiation potential; therefore, they are an attractive seeding source for tissue engineering therapy. Here we found that hMSCs with a scaffold did not affect cell viability and osteogenic differentiation. We also investigated regenerative effect of hMSCs with the scaffold in a calvarial bone defect model. Formation of new bone was evaluated by micro-CT, histology and expression of osteogenic markers. The results clearly showed interesting evidence indicating that hMSCs with scaffold increased the formation of new bone and expression of osteogenic markers, compared to the empty and scaffold only groups. Overall, our results suggest that hMSCs with scaffold are suitable for stimulation of intense bone regeneration in critical-sized bone defects.
Repair of bone defects remains a major clinical orthopedic challenge, in which autologous grafts and alloplastic materials were considered the gold standard. However, these procedures may cause donor-site morbidity, such as hemorrhage, infection, and chronic pain [1-3]. Recently, in an effort to overcome the problems, there has been a drive to engineer scaffolds that have the ability to incorporate host bone and provide structural support for cells during autologous tissue regeneration.
Scaffolds have the theoretical benefit of stimulation of cell attachment and proliferation while potentially serving as carriers for osteoinductive agents and osteoprogenitor cells [4,5]. Among the materials for scaffold, calcium phosphate has been widely used as bone scaffolds because of its inorganic nature and similarity to bone tissue [6]. Among cells with therapeutic potential, mesenchymal stem cells (MSCs) have gained significant interest as autologous cells, due to their capacity for self-renewal and differentiation into several types of mesenchymal tissues, including cartilage, adipose tissue, and bone [1,3,7]. In addition, treatment of bone defects using MSCs or genetically modified MSCs can effectively promote bone regeneration in human and animal models [7].
Based on these concepts and findings, this study was designed to determine whether human hMSCs with scaffold may support osteogenic differentiation and consequent bone regeneration. In this study, we show that MSCs with scaffold promote bone formation and functional osteogenic differentiation in critical-sized mouse calvarial bone defect.
We used 60 hydroxyapatite/40 β-Tricalcium phosphate for scaffold material. The scaffolds (Ossgen, Gyeongbuk Technopark, Gyeongbuk, Korea) were prepared as cylinders (4×2 mm) and have a porosity of 70%. The pore diameter was about 250-450 µm. The procedure for preparation of MSC was performed under Good Manufacturing Practice conditions at the Cell Therapy Center of Severance Hospital, Yonsei University, using a previously described method [8]. Bone marrow aspirates were prepared and mononuclear cells were isolated by Ficoll density centrifugation. Mononuclear cells were placed in 10-cm dishes. These cells were cultivated in low-glucose DMEM (Gibco), containing 10% FBS and 1% penicillin/streptomycin, and incubated in a humidified incubator at 37℃ in 5% CO2. Medium containing nonadherent cells was replaced every three days of culture. When the cells reached 70-80% confluence, they were trypsinized and subcultured. At passage 6 of the hMSCs, cells were seeded in scaffold and then transplanted into the calvarial defect.
Nonhealing, critical-sized (4 mm) calvarial defects were created in the right parietal bone of adult (60-day-old) male nude mice (BALB/c Sl c-nu/nu) using a dental drill. For defects considered acute, a scaffold was placed immediately at the time of injury. Osseous defects established were left empty, having only their skin incision closed following creation of bone defect. Mice were followed for eight weeks to ensure by micro-CT. In preparation for cell engraftment, scaffolds were seeded with hMSC. Briefly, 150,000 cells were resuspended in 25 mL of growth medium and seeded on the scaffold (OssGen) for 30 min. The scaffolds were subsequently submerged in 100 µL of growth medium for 48 h incubation. Animals were divided equally into three treatment groups: (1) empty defects, in which a 4-mm defect was created and left empty (n=10); (2) scaffold only, in which a scaffold without cells was placed in the defect site (n=10); (3) hMSCs with scaffold, in which hMSCs were impregnated in a scaffold and then placed in the defect site (n=10). Finally, the skin was sutured with 4-0 vicryl and the animal was monitored per established postoperative animal care protocols. Thereafter, animals were observed once daily for three days and weekly thereafter to ensure postoperative recovery. Mice were housed in a room maintained under controlled temperature and humidity on a 12 h/12 h light/dark cycle. All procedures were done in accordance with an animal protocol approved by the Kyungpook National University Institutional Animal Care and Use Committee (IACUC, KNU 2011-64).
Analysis of cell proliferation was performed using the WST-1 assay (Roche) according to the manufacturer's protocol. Briefly, hMSCs at a density of 1×103 cells/well were seeded into sterile 96-well plates. After performance of the differentiation procedures, the cells were incubated for an additional 48 h. WST-1 solution was then added to each well, and the cells were further incubated. After 4 h, the absorbance was measured in a microplate reader (Biochorm) at 450/655 nm and the data were expressed as a percentage of control.
In order to verify that hMSCs were capable of osteogenic differentiation in in vitro assays, hMSCs seeded with or without scaffolds were plated in 24-well plates at a density of 50,000 cells/well. Attachment cells were treated with osteogenic differentiation media (stem cell) supplemented with 1% penicillin/streptomycin. Medium was changed every three days. After seven days of differentiation induction, cells were fixed with a solution of 60% acetone and 40% citrate. After briefly washing with water, cells were stained with a diazonium salt solution composed of fast violet blue salt and 4% naphthol AS-MX phosphate alkaline solution. Alkaline phosphatase-positive cells were stained purple/red (10×objective).
Eight weeks post-surgery, mice were sacriced and the skulls were xed in 4% paraformaldehyde for 24 h at 4℃ and analyzed using X-eye micro-CT equipment (SEC, Korea), with a maximum tube current of 0.2 MA, a maximum tube voltage of 160 kV, and a focus size of 1 µm. The specimens were scanned through 360° at a spatial resolution of 30 µm. Files were reconstructed using a modied Feldkamp algorithm, which was created using microtomographic analysis software (i-cat 3D). Following the 3D visualization process, bone volumes were measured in the region of interest from three animals in each group. In addition, micro-CT scan was also performed in animals in which defects were not treated with any scaffolds, serving as the negative control. The bone volume was determined by subtracting the value of the negative control from the value of the experimental specimen.
Histology was performed using a modification of the Movat's Pentachrome and Aniline Blue stains, as previously described. At eight weeks, animals were sacrificed for histology. Calvariae were harvested, fixed in formalin, decalcified in 19% EDTA, and embedded in paraffin. Aniline blue staining was performed for identification of new osteoid matrix within the injury site, which was then analyzed by histomorphometry for quantification of new bone formation. Next, selected slides were stained with Pentachrome, in which bone appears bright yellow. Osteoclasts were identified by staining for tartrate-resistant acid phosphatase (TRAP). In brief, sections were stained using 225 µM Naphthol AS-MX phosphate (Sigma-Aldrich, St. Louis, MO, USA), 0.84% N,N-dimethylformamide (Sigma-Aldrich), and 1.33 mM Fast Red Violet LB Salt (Sigma-Aldrich) in 50 mM sodium acetate (pH 5.0) containing 50 mM sodium tartrate, and incubated for 30 min. Following incubation, sections were washed in distilled water and counterstained with 1% methyl green. TRAP activity appears as purplish to dark red staining within the cytoplasm of osteoclasts (4×20× objective).
The RNeasy Lipid Tissue Mini kit (Qiagen, Korea, Ltd.) was used according to the manufacturer's instructions for extraction of RNA. RNA samples from a total of three individual animals per experimental group were used for preparation of cDNA for RT-PCR using oligo (dT) primers and SuperScript III RT (Invitrogen). The QuantiTect SYBR Green PCR Kit (Qiagen, Korea, Ltd) was used for quantification of cDNA. PCR primers were as follows: Human Runt-related transcription factor 2 (RUNX2) (Forward 5'-ATTCCTGTAGATCCGAGCACC-3', Reverse 5'-GCTCACGTCGCTCATTTTGC-3'), Osteocalcin (Forward 5'-GGGCAATAAGGTAGTGAACAG-3', Reverse 5'-GCAGCACAGGTCCTAAATAG T-3'), Runt-related transcription factor 2 (RUNX2) (Forward 5'-ATACTGGGATGAGGAATGCG-3', Reverse 5'-CCAAGAAGGCACAGACAGAA-3'), GAPDH (Forward 5'-TGTGAGGGAGATGCTCAGTG-3', Reverse 5'-GTGGACCTCATGGCCTACAT-3'). For each investigated transcript, a mixture of the following reaction components was prepared according to the indicated end concentration: forward primer (5 pM), reverse primer (5 pM) and QuantiTect SYBR Green PCR Master mix; 10 mL of master mix was added to a 0.1 mL tube and a 5 mL volume, containing 100 ng reverse transcribed total RNA, was added as the PCR template. Quantitative real-time PCR was performed using a Corbett Rotor-Gene 6000 quantitative PCR system (Corbett Life Sciences, Sydney, Australia) with the following cycling parameters: 95℃ for 15 min followed by 40 cycles of 95℃ for 10 sec, 58℃ for 15 sec, 72℃ for 20 sec. The two delta delta CT method was used to determine mean fold changes in gene expression between the control and target genes. The results were normalized to GAPDH expression.
The Student's t-test was used for comparison of two groups, whereas Tukey's HSD test and Repeated Measures Analysis of Variance test was used for multi group comparisons according to the SAS statistical package (release 9.1; SAS Institute Inc., Cary, NC). P<0.05 was considered significant.
The effects of scaffold on in vitro cell viability and osteogenic differentiation were assessed. The number of viable cells was measured using the WST-1 assay. The results showed no significant differences in cell viability of hMSCs with or without scaffold (Figure 1A). Next, we inquired as to the more relevant influence of scaffold in osteogenic differentiation of hMSCs. Alkaline phosphatase enzymatic activity, which appears as a purple stain, and is representative of early osteogenic differentiation, was assessed at seven days differentiation. The results also showed no significant differences in osteogenic differentiation of hMSCs with or without scaffold (Figure 1B,1C). These results demonstrate that scaffold does not affect cell viability and osteogenic differentiation.
To evaluate the potential of hMSCs with scaffold for bone regeneration in vivo, 4-mm bone defects were created in the calvarial bones of 60-day old nude mice. The experimental design is described in Figure 2A. Three experimental groups were assayed at eight weeks post calvarial defect. This critical-sized calvarial defect cannot heal spontaneously during the bone healing period [7]. The cranial bones were harvested at eight weeks after creation of bone defects. Photographs after eight weeks confirmed a near complete lack of healing in empty defects. In marked contrast, defects treated with hMSCs with scaffold showed robust bone regeneration (Figure 2B). For assessment of bone regeneration, we performed micro-CT analysis at eight weeks post creation of bone defects. The empty group showed little or no healing by micro-CT. The hMSCs with scaffold group showed greater healing than the scaffold only group (Figure 2C). Quantification of micro-CT images was performed next and evaluated according to percentage healing of the defects. The empty defect group showed healing of less than 10% over the course from creation of bone defects. The scaffold only group showed a significant increase in healing percentage, amounting to 80% after creation of bone defects. Finally, the hMSCs with scaffold group showed greater healing by approximately 90% than the scaffold only group after creation of bone defects (Figure 2D). These results demonstrate successful healing of a calvarial defect treated with hMSCs in scaffold.
For observation of new bone formation in the reconstructed defects, aniline blue and pentachrome staining were performed on calvarial defects at eight weeks after injury. Aniline blue staining was used for identification of new osteoid matrix within the calvarial defect site, in which bone appears dark blue. The hMSCs with scaffold and scaffold only groups showed significant aniline blue-positive bone formation within the defect site, compared with the empty group. Adjacent slides were stained with pentachrome, in which bone muscle appears red. hMSCs with scaffold showed significant red staining within the defect site, higher than that of scaffold only (Figure 3A). The amount of osteoid matrix was quantified. Results of this quantication indicated that the hMSCs with scaffold group exhibited signicantly more bone formation than the empty or scaffold only groups (Figure 3B). The lack of healing bone regeneration in the empty group may be due to differences in osteoclasts. Therefore, we performed TRAP staining. No osteoclast-specic staining could be detected within each of our groups, suggesting that the observed extent of bone formation was not secondarily modulated by osteoclast-mediated bone resorption (Figure 3C).
To further investigate the question of whether hMSCs show osteogenic differentiation, human (h) RUNX2, mouse (m) RUNX2, and mouse (m) Osteocalcin were analyzed by real-time PCR. The results showed differentiation of mRUNX2 and mOsteocalcin, which were expressed from one to two weeks (Figure 3D). These results indicate that hMSCs stimulate osteogenic factors in vivo during a time period corresponding to calvarial defects, leading to supportive osteogenic differentiation of hMSCs with scaffold.
The combination of tissue engineering and stem cell therapy is a promising strategy for bone regeneration. Many studies have reported on the bone regenerating capacity of MSCs [1,3,9]. Some studies have shown that scaffolds should provide sufficient mechanical support, osteogenic differentiation and consequent bone formation in vivo [2,7]. Combination of scaffold and MSCs resulted in more rapid bone regeneration and applied clinically for treatment of bone defects. Here we have produced a calcium phosphate scaffold material (HA/β-TCP) intended for tissue engineering. We also used medical grade high purity nano size calcium phosphate powders and high sintering density with nano-sized spherical particle shape for scaffolds material. Comparing to organic scaffold material, such as a collagen and gelatin scaffold, nonorganic scaffold material including calcium phosphate cement (CPC), β-TCP, usually possesses sufficient mechanical strength [6].
This study investigated a therapeutic strategy using hMSCs with scaffold in healing a critical-sized calvarial defect with scaffold. In our study, we attempted to determine whether hMSCs grafted onto a scaffold can support osteogenesis mediated by co-implanted MSCs in a critical-sized calvarial defect model of nude mice. According to the results of our study, hMSCs showed good attachment to the scaffolds. After 48 h incubation, WST-1 assay showed that adhesion, proliferation, and viability of hMSCs for the scaffold was not affected compared with cells alone. Consistent with a previous study [6], treatment with scaffold resulted in significantly enhanced hMSCs proliferation, osteogenic differentiation, and bone formation when compared with cells alone.
Some studies have suggested that MSCs are ideal seed cells for bone formation. MSCs are expected to be beneficial when used in multipotent stem cells for bone regeneration since they are related to certain factors, including VEGF, BMP, and Runx2 [6,10-13]. In our experiments, we suggest that acceleration of bone defect healing by hMSCs with scaffold is due to an increase in the expression levels of mRunx2 and mOsteocalcin. Osteocalcin is mainly secreted by mature osteoblasts at the late stage of osteoblastic differentiation [13].
Also in our study, quantitation analysis by micro-CT revealed that bone fracture healing in the hMSCs with scaffold group was increased, compared with that in the control. Histological examination demonstrated an increase in the percentage of new bone area in the hMSCs with scaffold group, compared with the empty group. Thus, hMSCs with scaffold can effectively produce new bone in bone defect in vivo.
In summary, findings of this study demonstrated that hMSCs with scaffold are a readily available cell source capable of osteogenesis for healing of critical-sized mouse calvarial defects. These results may be beneficial to stem cell-based therapies for clinical implementation. Findings of our study also indicated that the scaffold can support proliferation and viability of hMSCs for clinical applications [14]. Incorporation of hMSCs with scaffold is a stimulator of osteoblast marker expression and improves bone regeneration.
Acknowledgments
This work was supported by Kyungpook National University Research Fund (2012). Additional support for this work was provided by Leading Industry Development for Economic Region (2009-T-2-A-Y0-A-05) funded by the Ministry of Knowledge Economy (MKE) and Basic Science Research Program through the National Research Foundation of Korea (NRF) funded by the Ministry of Education, Science and Technology (2013R1A1A20082963), Korea.
References
1. Koob S, Torio-Padron N, Stark GB, Hannig C, Stankovic Z, Finkenzeller G. Bone formation and neovascularization mediated by mesenchymal stem cells and endothelial cells in critical-sized calvarial defects. Tissue Eng Part A. 2011; 17(3-4):311–321. PMID: 20799886.


2. Levi B, James AW, Nelson ER, Vistnes D, Wu B, Lee M, Gupta A, Longaker MT. Human adipose derived stromal cells heal critical size mouse calvarial defects. PLoS One. 2010; 5(6):e11177. PMID: 20567510.


3. Zong C, Xue D, Yuan W, Wang W, Shen D, Tong X, Shi D, Liu L, Zheng Q, Gao C, Wang J. Reconstruction of rat calvarial defects with human mesenchymal stem cells and osteoblast-like cells in poly-lactic-co-glycolic acid scaffolds. Eur Cell Mater. 2010; 20:109–120. PMID: 21249628.


4. Amorosa LF, Lee CH, Aydemir AB, Nizami S, Hsu A, Patel NR, Gardner TR, Navalgund A, Kim DG, Park SH, Mao JJ, Lee FY. Physiologic load-bearing characteristics of autografts, allografts, and polymer-based scaffolds in a critical sized segmental defect of long bone: an experimental study. Int J Nanomedicine. 2013; 8:1637–1643. PMID: 23637532.


5. Xing Z, Xue Y, Dånmark S, Schander K, Ostvold S, Arvidson K, Hellem S, Finne-Wistrand A, Albertsson AC, Mustafa K. Effect of endothelial cells on bone regeneration using poly(L-lactide-co-1,5-dioxepan-2-one) scaffolds. J Biomed Mater Res A. 2011; 96(2):349–357. PMID: 21171154.
6. Zou D, Zhang Z, He J, Zhu S, Wang S, Zhang W, Zhou J, Xu Y, Huang Y, Wang Y, Han W, Zhou Y, Wang S, You S, Jiang X, Huang Y. Repairing critical-sized calvarial defects with BMSCs modified by a constitutively active form of hypoxia-inducible factor-1 and a phosphate cement scaffold. Biomaterials. 2011; 32(36):9707–9718. PMID: 21975460.


7. He X, Dziak R, Yuan X, Mao K, Genco R, Swihart M, Sarkar D, Li C, Wang C, Lu L, Andreadis S, Yang S. BMP2 genetically engineered MSCs and EPCs promote vascularized bone regeneration in rat critical-sized calvarial bone defects. PLoS One. 2013; 8(4):e60473. PMID: 23565253.


8. Lee PH, Kim JW, Bang OY, Ahn YH, Joo IS, Huh K. Autologous mesenchymal stem cell therapy delays the progression of neurological deficits in patients with multiple system atrophy. Clin Pharmacol Ther. 2008; 83(5):723–730. PMID: 17898702.


9. Qi Y, Du Y, Li W, Dai X, Zhao T, Yan W. Cartilage repair using mesenchymal stem cell (MSC) sheet and MSCs-loaded bilayer PLGA scaffold in a rabbit model. Knee Surg Sports Traumatol Arthrosc. 2012.


10. Levi B, James AW, Nelson ER, Peng M, Wan DC, Commons GW, Lee M, Wu B, Longaker MT. Acute skeletal injury is necessary for human adipose-derived stromal cell-mediated calvarial regeneration. Plast Reconstr Surg. 2011; 127(3):1118–1129. PMID: 21364415.


11. Huang S, Wang Z. Platelet-rich plasma-derived growth factors promote osteogenic differentiation of rat muscle satellite cells: in vitro and in vivo studies. Cell Biol Int. 2012; 36(12):1195–1205. PMID: 22988823.
12. Faghihi F, Baghaban Eslaminejad M. The effect of nano-scale topography on osteogenic differentiation of mesenchymal stem cells. Biomed Pap Med Fac Univ Palacky Olomouc Czech Repub. 2013.


13. Wang L, Rao RR, Stegemann JP. Delivery of mesenchymal stem cells in chitosan/collagen microbeads for orthopedic tissue repair. Cells Tissues Organs. 2013; 197(5):333–343. PMID: 23571151.


14. Rampichová M, Chvojka J, Buzgo M, Prosecká E, Mikeš P, Vysloužilová L, Tvrdík D, Kochová P, Gregor T, Lukáš D, Amler E. Elastic three-dimensional poly (-caprolactone) nanofibre scaffold enhances migration, proliferation and osteogenic differentiation of mesenchymal stem cells. Cell Prolif. 2013; 46(1):23–37. PMID: 23216517.


Figure 1
Viability and differentiation of hMSCs. (A) WST-1 assay was performed on the different scaffolds after 48 h in culture. No differences were observed in cell viability with or without scaffolds. (B,C) Alkaline phosphatase staining, hMSCs with or without scaffold undergo in vitro osteogenic differentiation. Scale bar, 100 mm. Data are expressed as mean±SEM (Student's t-test,*P<0.05 compared with hMSC).
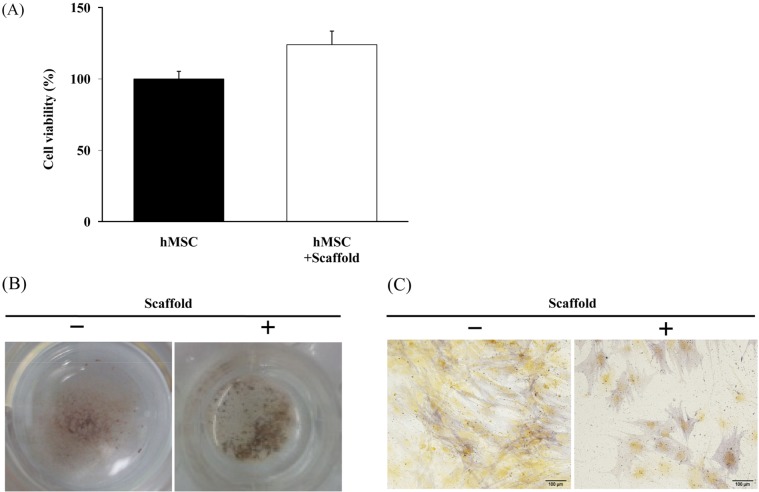
Figure 2
Differences in osteogenic healing of calvarial defects. (A) Timeline of the experimental design of this study. (B) Four millimeter calvarial defects were created in the parietal bone of nude mice. Treatment groups included empty defects, defects treated with scaffold only, or bone defects treated hMSCs with scaffold (empty group [n=10]; scaffold only group [n=10]; hMSCs + scaffold group [n=10]). Instead, a new layer of woven bone healed the critical-sized defect. Dashed lines encircle the original defect. (C) Micro-CT scanning at eight weeks revealed near complete lack of healing among empty defects. Near healing of hMSCs with scaffold was observed within eight weeks. (D) Quantification of Micro-CT. At eight weeks, healing of defects represented as a fraction of total defect area was quantified by micro-CT images. (ANOVA, Tukey's HSD test, *P<0.005 compared with empty defects group).
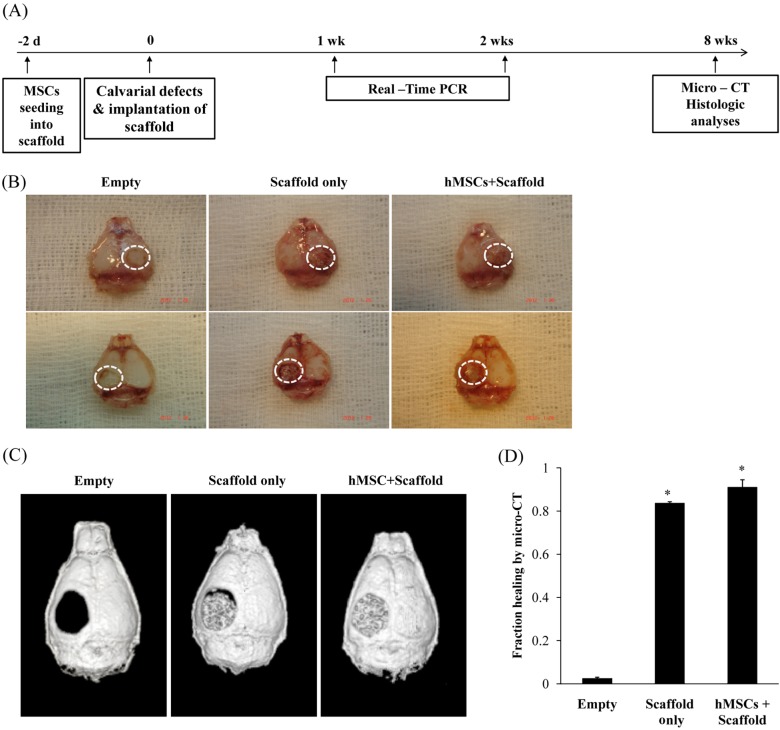
Figure 3
Histologic analysis of bone formation. Serial sections throughout the defect site were created at eight weeks post injury. Defects shown include those empty, those treated with scaffold only immediately after creation of the defect, and those treated with hMSCs with scaffold at eight weeks after creation of the calvarial defect. (A) Stains included aniline blue and pentachrome. (B) At eight weeks, aniline blue-positive bone per 4.0×, 20.0× field was quantified. (C) TRAP staining. TRAP staining was performed for determination of the amount of osteoclasts within the explants from the reconstructed defects. No positive TRAP staining (should be indicated by red color) could be detected within the explants. (D) Osteogenic gene expression by Real-Time PCR at one and two weeks post-injury among hMSC engrafted defects, including hRUNX2, mRUNX2, and mOsteocacin. Data are expressed as mean±SD from four independent experiments (Anova, Tukey's HSD test, *P<0.005 compared with empty defects group). Scale bar, 100 mm, 500 mm.
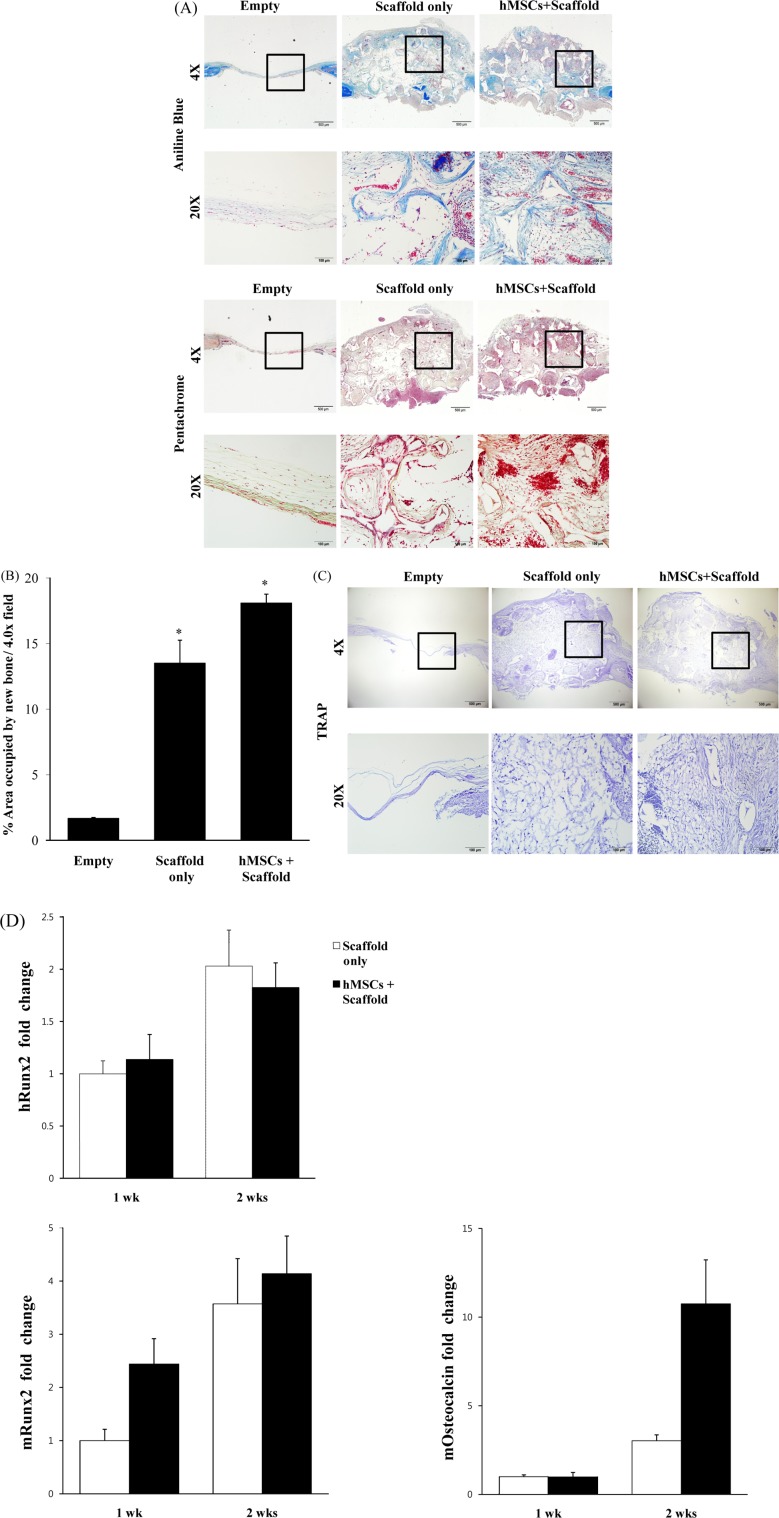