Abstract
Muscle atrophy is the result of two opposing conditions that can be found in pathological or diseased muscles: an imbalance in protein synthesis and degradation mechanisms. Thus, we investigated whether exogenous melatonin could regulate muscle components in stroke-induced muscle atrophy in rats. Comparing muscle phenotypes, we found that long-term melatonin administration could influence muscle mass. Muscle atrophy-related genes, including muscle atrophy F-box (MAFbx) and muscle ring finger 1 (MuRF1) were significantly down-regulated in melatonin-administered rats in the gastrocnemius. However, only MAFbx at the mRNA level was attenuated in the soleus of melatonin-administered rats. Insulin-like growth factor-1 receptor (IGF-1R) was significantly over-expressed in melatonin-administered rats in both the gastrocnemius and soleus muscles. Comparing myosin heavy chain (MHC) components, in the gastrocnemius, expression of both slow- and fast-type isoforms were significantly enhanced in melatonin-administered rats. These results suggest that long-term exogenous melatonin-administration may have a prophylactic effect on muscle atrophy through the MuRF1/MAFbx signaling pathway, as well as a potential therapeutic effect on muscle atrophy through the IGF-1-mediated hypertrophic signaling pathway in a stroke animal model.
Skeletal muscle atrophy is a change that can occurs in the muscles of adult animals as a result of several causes, such as disuse (e.g., immobilization, denervation, space flight), starvation, and some diseases [1-5]. Additionally, muscle atrophy can take place secondary to some devastating injuries or common health problems, such as injuries of the central nervous system (e.g., stroke, spinal cord injury), aging, and various systemic diseases [1].
Muscle atrophy is the result of an imbalance between two opposing conditions that can occur in pathological or diseased muscles: an imbalance in protein synthesis and degradation mechanisms [6-9]. Atrophy is characterized by wasting or loss of muscle mass and usually involves a decrease in the size or cross-sectional area (CSA) of individual myofibers [8,9]. Previous studies demonstrated that the slow-twitch fibers of the extensor muscles in young rats during space flight (SF)-induced atrophy were particularly sensitive to atrophy [1,3]. Paretic muscle atrophy, associated with acute brain injury, is characterized by the appearance of variability in fiber diameter, nuclear internalization, and perifascicular fatty infiltration [11].
Inflammation is a fundamental pathogenic mechanism in stroke. Elevated inflammatory markers predict risk for incident and recurrent stroke [10]. Tumor necrosis factor-α (TNF-α), an inflammatory cytokine, is expressed at negligible levels in normal muscle but is elevated under selected metabolic conditions characterized by muscle wasting and insulin resistance [9,10]. Thus, previous studies have reported that the expression of TNF-α mRNA is increased in the skeletal muscle of stroke patients [7,10,12]. TNF-α may contribute to atrophy through a number of mechanisms, including inhibition of protein synthesis, transcriptional regulation of myofiber gene expression, and accelerated protein breakdown through activation of ubiquitin proteases, nuclear factor kappa-light-chain-enhancer of activated B cells (NF-κB), and apoptotic cell death [10,13]. TNF-α appears to preferentially down-regulate MHC type I protein synthesis and enhance its degradation. However, it elevated MHC type II in paretic muscles [10,11].
Numerous studies have demonstrated that overexpression of NF-κB in disuse conditions activates the machinery of MAFbx/MuRF1 signaling [6,10]. These genes are expressed only in skeletal muscle and myocardium and have been found to be expressed at elevated levels under all conditions involving atrophy, including denervation [10,13,14]. Over-expression of MuRF1 reduces the size of cultured muscle cells, and MAFbx targets the myosin heavy chain for degradation [9]. A protein imbalance in skeletal muscle activates the PI3K/Akt/mTOR signaling pathway. Phosphatidylinositol 3-kinases (PI3K) activate Akt, leading to reduced tuberous sclerosis complex (TSC1/2) and forkhead box (FOXO) protein. The TSC1/2 protein inhibits mammalian target of rapamycin (mTOR) signaling, and once TSC1/2 is reduced, mTOR increases, increasing expression of p70S6K in ribosomes [8,9].
IGF-1 is a major upstream molecule in muscle hypertrophy. Thus, the recovery machinery is activated, followed by expression of IGF-1 [5,6,14]. Other studies reported that IGF-1 induced activation of the PI3K/Akt/mTOR signal pathway, increasing the proliferation of satellite cells [14-16].
Administration of IGF-1 is mitogenic for myoblasts, increases muscle protein content, and reduces protein degradation [14]. More importantly, over-expression of IGF-1 has been associated with the proliferation of satellite cells [14,16] when injected into an acute contusion, laceration, or strained muscle, increasing regeneration [14,16,17]. IGF-1 not only inhibits atrophy signaling but also activates the PI3K/Akt/mTOR signaling pathway [14,17,18].
Melatonin, the major secretory product of the pineal gland, is known to interact with the neuroendocrine axis and circadian rhythms [19-23]. In a previous study, we demonstrated that exogenous and endogenous melatonin has a prophylactic effect on muscle atrophy in the hindlimb in spinal cord-injured rats [20]. In vivo studies have shown that melatonin treatment increases the expression of IGF-1 in a castration animal model [21]. Thus, in this study, we investigated whether exogenous melatonin could regulate muscle components in stroke-induced muscle atrophy in rats.
All animal procedures were approved by the Ethics Committee for Animal Care and Use at Inje University (Approval No. 2010-21). The rats were randomly divided the control (Con), and MCAo groups (Veh: MCAo+vehicle, MT7: MCAo+melatonin injection at 7:00, MT19: MCAo+melatonin injection at 19:00, and MT7,19: MCAo+melatonin injection at 7:00 and 19:00). Focal cerebral ischemia was induced by a modified intraluminal occlusion method, as described previously [22,23]. Briefly, the junction of right common carotid artery was exposed under operating microscope. A 4-0 Nylon thread, with its tip blunted by heating over flame, was inserted 18.5±1.0 mm from external into the internal carotid artery until the tip occluded the origin of the MCA. After closure of the operative sites, the animals were temporarily transferred to a cage with a heating lamp. The thread was gently removed at 60 min of MCA occlusion. Melatonin (Sigma-Aldrich, St Louis, MO, USA) was dissolved in a mixture of EtOH and 0.9% physiological saline (final concentration, 5%). Fresh melatonin solution was prepared in a dark room with switch on the red lamp. Animals were injected subcutaneously melatonin (10 mg/kg) or Veh (EtOH-0.9% physiological saline) at 7:00 and 19:00 from 24 hr after MCAo. Neurological deficiency tests were conducted as described previously [22].
The animals were sacrificed after anesthetized by cocktail of zoletil (40 mg/kg) with xylazine (10 mg/kg). The fresh skeletal muscle tissues were homogenized with 1ml of Tri-reagent (Sigma-Aldrich, St. Louis, MO, USA) to prepare a total RNA samples. The RNA was reverse transcribed with oligo d(T) 12~18 using reverse transcriptase #18064-014 (Invitrogen, Carlsbad, CA, USA) and this reaction mixture served as a template for polymerase chain reaction (PCR). To identify gene transcription, a reaction mixture (50 µL) for PCR was made up of 2.0 µL of cDNA synthesis mixture, 40 nM dNTPs, 10 pM of sense and antisense primer, and 1.25 U of GoTaq® DNA polymerase (Promega, Medison, WI, USA). PCR were performed with denaturation at 95℃ for 30 sec, annealing at 60℃ for 1 min, and extension at 72℃ for 1min in each cycle, followed by a final 10 min extension at 72℃ using Px2 Thermal cycler HBPX2220 (Thermo electron corporation, Waltham, MA, USA). Primer sets are listed in Table 1. The histological study also used previously described methods for hematoxylin and eosin (H&E) staining [22].
Based on previous results, we assessed melatonin treatment and changes in hindlimb muscles. The MT19 group showed significantly attenuated muscle mass loss in both the right and left hindlimbs (P<0.01; Figure 1A). Similar results were observed in the soleus muscles (Figure 1B). However, MT7,19 rats showed no difference in the gastrocnemius muscle compared with the Con, indicating recovery of muscle mass following melatonin treatment (Figure 1A). From the histological study, long-term melatonin administration may enhance the volume of muscle fibers. Interestingly, we also found a mild accumulation of fat between muscle fibers in the soleus of MT19 rats (Figure 2).
The regulation of muscle atrophy is important for rehabilitation therapeutic approaches in recovery-stage patients after a stroke. Briefly, the physiological condition of muscle tissues has to be appropriate for functional movement. Thus, we compared to atrophy-related gene expression in innervated hindlimb muscles following the time of melatonin administration in focal cerebral ischemic rats. In typical intermediate-type muscles, such as the gastrocnemius, the MAFbx and MuRF1 genes are significantly suppressed following long-term melatonin administration. In particular, MT7,19 rats showed a strong protective effect against stroke-induced muscle atrophy (P<0.01; Figure 3A). At the same time, melatonin turned on the hypertrophic signaling pathway through IGF-1R expression in the MT7,19 group (P<0.01, P<0.01; Figure 3A).
In a slow-twitch muscle, such as the soleus, MAFbx gene expression was significantly down-regulated by twice daily melatonin administration, at 7:00 and 19:00 (P<0.01, P<0.01; Figure 3B). However, MuRF1 was unaltered. IGF-1R gene expression was clearly enhanced in the soleus of MT7,19 rats (P<0.01, P<0.01; Figure 3B).
We measured whether exogenous melatonin caused changes in MHC components during recovery after stroke. In the gastrocnemius, all MHC components showed similar expression. After 8 weeks of treatment, Veh rat showed greater expression of MHC-IIb in fast-twitch type muscles compared with that in Con (P<0.01; Figure 4A). However, MT7 and MT7,19 rats showed up-regulated expression of all MHC components. Especially in the MT7,19 rat, MHC components were significantly enhanced in both slow- and fast-twitch muscle types after 8 weeks (P<0.01). MT7 rats showed a similar effect in the gastrocnemius (Figure 4A).
The results in the soleus were interesting. The soleus is a typical slow-twitch muscle in the hindlimb. We observed spontaneous restoration of MHC-Iβ components in stroke-induced muscle atrophy (Figure 4B), indicating that exogenous melatonin had a therapeutic effect against stroke-induced muscle atrophy.
In previous studies, we confirmed that melatonin could prevent oxidation-induced cell death as well as improve neurological function in focal cerebral ischemic rats [22,23]. We suggested that melatonin might be useful as a therapeutic agent for focal cerebral ischemia. However, stroke leads to atrophy of ipsilateral brain-innervated skeletal muscles. Thus, we analyzed atrophyrelated gene expression and changes in MHC isoforms.
Skeletal muscle function is fundamental for functional restoration in stroke patients [7,13]. Thus, clinical trials have examined the efficacy of treatments for the rehabilitation of neurological disorder patients. Skeletal muscle characterized the change of muscle type under physiological condition, and the existence of intrinsic differences between muscles that limit the range of possible adaptations by external environments [24]. Therefore, we examined whether exogenous melatonin had a beneficial effect in stroke-induced muscle atrophy in the gastrocnemius and soleus muscles. A recent study reported that TNF-α was elevated in paretic muscles in stroke patients [10]. TNF-α contributes to atrophy through a number of mechanisms, including inhibition of protein synthesis, transcriptional regulation of myofiber gene expression, and accelerated protein breakdown through activation of ubiquitin proteases and NF-κB. TNF-α appears to preferentially down-regulate MHC-type I protein synthesis and enhance its degradation. However, it elevated MHC-type II in the paretic muscle [9,10,14]. Previous studies demonstrated that the MuRF1 and MAFbx genes are major players in the muscle atrophy signaling pathway. Over-expression of MuRF1 reduces the size of cultured muscle cells, and MAFbx targets the myosin heavy chain for degradation [8,9]. Lin et al [25] reported that melatonin has not only beneficial effects to attenuate systemic inflammatory cytokines, such as TNF-α, IL-Iβ, and IL-6 but also promotes an antiinflammatory cytokine, including IL-10 on heatstroke-induced multiple organ dysfunction syndrome. We found that middle cerebral artery occlusion (MCAo) rats showed slightly enhanced expression of MAFbx/MuRF1 mRNA levels, and exogenous melatonin had a prophylactic and therapeutic effect during stroke-induced muscle atrophy. Exogenous melatonin has differential effects, depending on administration time. This may be related to the plasma concentration of melatonin. In a previous study, we confirmed that a controlled photoperiod could change plasma levels of melatonin [26]. Moreover, therapeutic effect of melatonin could modulate via both photoperiod control and exogenous melatonin administration on reconstruction of neural network after spinal cord injury in rats [20]. In clinical trials, likewise, melatonin administration (10 mg/day) induces reduction of progression of non-alcoholic fatty liver disease through improves plasma liver enzymes [27]. Thus, we examined whether melatonin levels in plasma affected physiological conditions. Our results suggest that melatonin might attenuate stroke-induced muscle atrophy by down-regulating the MAFbx/MuRF1 signaling pathway. Additionally, exogenous melatonin may be a possible candidate for hormonal therapy in stroke-induced muscle atrophy. Moreover, melatonin administration time might be an important factor for physiological effect of melatonin in vivo.
Protein imbalance in skeletal muscle activates the PI3K/Akt/mTOR signaling pathway [1,4,8]. When PI3K is activated by IGF-1, it reduces the levels of TSC1/2 and FOXO proteins. TSC1/2 inhibits the mTOR signaling pathway. Consequently, when TSC1/2 is decreased, mTOR increases protein synthesis in ribosomes. IGF-1 is an upstream molecule in the PI3K/Akt/mTOR signaling pathway. Another study reported that exogenous IGF-1 increased the proliferation of satellite cells in skeletal muscle [13-15]. Administration of IGF-1 increased muscle protein content and down-regulated protein breakdown [14,21]. We demonstrated that exogenous melatonin could activate the transcription of IGF-1 mRNA. This means that IGF-1R is turned on in downstream signaling pathways, such as the PI3K/Akt/mTOR signaling pathway.
Here, we suggest that the therapeutic effect of melatonin might have different physiological effects following the time of melatonin administration. At this point, we considered the re-arrangement of MHC components in muscle types.
We next analyzed the expression of MHC mRNA levels in the gastrocnemius and soleus. The gastrocnemius muscle is a well-known intermediate-type muscle, and its properties can be affected by changes in the extrinsic environment [13]. However, there was not altered the muscle phenotype in gastrocnemius after focal cerebral ischemia. We found similar results with MAFbx and MuRF1 gene expression. MAFbx was not significantly altered in the gastrocnemius. In contrast, MuRF1 was significantly activated at the mRNA level in the gastrocnemius of Veh rats, suggesting that stroke-induced muscle atrophy involves non-specific protein degradation in the gastrocnemius. Additionally, all of MHC isoforms were significantly observed an increment of mRNA level in the MT7 and MT7,19 rats. The soleus is a typical slow-twitch muscle. The MAFbx mRNA level was slightly increased in the soleus in the Veh rats and significantly down-regulated in MT7,19 rats (P<0.01), indicating that the soleus showed reduction in muscle fiber size after stroke, and protein degradation could be reduced by exogenous melatonin. Comparing the MHC components in the gastrocnemius, MT7 and MT7,19 rats showed significantly increased MHC-Iβ (P<0.05), MHC-IIa (P<0.01), and IIx (P<0.01). In the soleus, all MHC components were significantly up-regulated by melatonin administration at 7:00 (MHC-IIb: P<0.05; MHC-Iβ, -IIa, IIx: P<0.01). The gastrocnemius and soleus muscles showed only loss of the MHC-IIx isoform; the other isoforms of MHC were augmented by exogenous melatonin. This indicates that in stroke-induced atrophy, a slow-twitch muscle might transform to a fast-twitch muscle. On the other hands, the soleus might not be affected by stroke. We also found that melatonin could induce protein synthesis. Accordingly, stroke-induce muscle atrophy leads to break down the MHC of fast type in hindlimb muscles, and is turned on the recovery machinery for protein synthesis followed by melatonin administrating time-point. The limitation of this study, further investigation of the appropriate mechanism related with the restoration of neurological function and recovery of muscle atrophy following melatonin administration is required. In here, we suggest that melatonin might have physiological effects which might be diverse following administrating time-points. In conclusion, these results suggest that long-term exogenous melatonin treatment might have a prophylactic effect against muscle atrophy through the MuRF1/MAFbx signaling pathway, as well as a therapeutic effect in muscle atrophy via the IGF-1-mediated hypertrophic signaling pathway in a stroke animal model.
Figures and Tables
Figure 1
Changes in muscle mass following melatonin administration for 8 weeks after MCAo. (A) Gastrocnemius, (B) Soleus. Right means to sound side hindlimb. Left means to affected side hindlimb. Rt: right hindlimb; Lt: left hindlimb; Con: control; Veh: MCAo+vehicle; MT7: MCAo+melatonin injection at 7:00; MT19: MCAo+melatonin injection at 19:00; MT7,19: MCAo+melatonin injection at 7:00 and 19:00. **P<0.01 vs. Con.
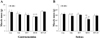
Figure 2
Morphology of hindlimb muscles following melatonin administration for 8 weeks after MCAo. Veh: MCAo+vehicle; MT7: MCAo+melatonin injection at 7:00; MT19: MCAo+ melatonin injection at 19:00; MT7,19: MCAo+melatonin injection at 7:00 and 19:00. Scale bar=100 µm.
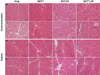
Figure 3
Changes of muscle atrophy- and hypertrophy-related gene expression on stroke-induced muscle atrophy in the gastrocnemius (A) and soleus (B). MT7,19 rats showed inhibition of both MAFbx and MuRF1 mRNA expression and an increase in hypertrophic upstream molecules, such as IGF-1R expression. Con: control; Veh: MCAo+vehicle; MT7: MCAo+ melatonin injection at 7:00; MT19: MCAo+melatonin injection at 19:00; MT7,19: MCAo+melatonin injection at 7:00 and 19:00. (A) **P<0.01 vs. Con; ##P<0.01 vs. Veh. (B) *P<0.05, **P<0.01 vs. Con; #P<0.05, ##P<0.01 vs. Veh.
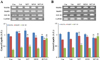
Figure 4
Changes of MHC components on stroke-induced muscle atrophy in the gastrocnemius (A) and soleus (B). MT7 and MT7,19 rats showed increases in both slow- and fast-type MHC isoforms. Con: control; Veh: MCAo+vehicle; MT7: MCAo+melatonin injection at 7:00; MT19: MCAo+melatonin injection at 19:00; MT7,19: MCAo+melatonin injection at 7:00 and 19:00. (A) *P<0.05, **P<0.01 vs. Con; #P<0.05, ##P<0.01 vs. Veh. (B) *P<0.05, **P<0.01 vs. Con; #P<0.05, ##P<0.01 vs. Veh.
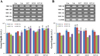
Acknowledgment
The study was funded by the KRIBB Research Initiative Program (KGM0501113 to Y. Hong), Republic of Korea.
References
1. Jackman RW, Kandarian SC. The molecular basis of skeletal muscle atrophy. Am J Physiol Cell Physiol. 2004. 287(4):C834–C843.
2. Kasper CE, Talbot LA, Gaines JM. Skeletal muscle damage and recovery. AACN Clin Issues. 2002. 13(2):237–247.
3. Tischler ME, Henriksen EJ, Munoz KA, Stump CS, Woodman CR, Kirby CR. Spaceflight on STS-48 and earth-based unweighting produce similar effects on skeletal muscle of young rats. J Appl Physiol. 1993. 74(5):2161–2165.
4. Zeman RJ, Zhao J, Zhang Y, Zhao W, Wen X, Wu Y, Pan J, Bauman WA, Cardozo C. Differential skeletal muscle gene expression after upper or lower motor neuron transection. Pflugers Arch. 2009. 458(3):525–535.
5. Drummond MJ, Glynn EL, Lujan HL, Dicarlo SE, Rasmussen BB. Gene and protein expression associated with protein synthesis and breakdown in paraplegic skeletal muscle. Muscle Nerve. 2008. 37(4):505–513.
6. Nader GA, Hornberger TA, Esser KA. Translational control: implications for skeletal muscle hypertrophy. Clin Orthop Relat Res. 2002. (403 Suppl):S178–S187.
7. Hafer-Macko CE, Ryan AS, Ivey FM, Macko RF. Skeletal muscle changes after hemiparetic stroke and potential beneficial effects of exercise intervention strategies. J Rehabil Res Dev. 2008. 45(2):261–272.
8. Boonyarom O, Inui K. Atrophy and hypertrophy of skeletal muscles: structural and functional aspects. Acta Physiol (Oxf). 2006. 188(2):77–89.
9. McKinnell IW, Rudnicki MA. Molecular mechanisms of muscle atrophy. Cell. 2004. 119(7):907–910.
10. Hafer-Macko CE, Yu S, Ryan AS, Ivey FM, Macko RF. Elevated tumor necrosis factor-alpha in skeletal muscle after stroke. Stroke. 2005. 36(9):2021–2023.
11. Scelsi R, Lotta S, Lommi G, Poggi P, Marchetti C. Hemiplegic atrophy: Morphological findings in the anterior tibial muscle of patients with cerebral vascular accidents. Acta Neuropathol. 1984. 62(4):324–331.
12. Kernan WN, Inzucchi SE. Type 2 Diabetes Mellitus and Insulin Resistance: Stroke Prevention and Management. Curr Treat Options Neurol. 2004. 6(6):443–450.
13. De Deyne PG, Hafer-Macko CE, Ivey FM, Ryan AS, Macko RF. Muscle molecular phenotype after stroke is associated with gait speed. Muscle Nerve. 2004. 30(2):209–215.
14. Day CS, Buranapanitkit B, Riano FA, Tomaino MM, Somogyi G, Sotereanos DG, Kuroda R, Huard J. Insulin growth factor-1 decreases muscle atrophy following denervation. Microsurgery. 2002. 22(4):144–151.
15. Ryan AS, Dobrovolny CL, Smith GV, Silver KH, Macko RF. Hemiparetic muscle atrophy and increased intramuscular fat in stroke patients. Arch Phys Med Rehabil. 2002. 83(12):1703–1707.
16. Machida S, Booth FW. Insulin-like growth factor 1 and muscle growth: implication for satellite cell proliferation. Proc Nutr Soc. 2004. 63(2):337–340.
17. Barton-Davis ER, Shoturma DI, Musaro A, Rosenthal N, Sweeney HL. Viral mediated expression of insulin-like growth factor I blocks the aging-related loss of skeletal muscle function. Proc Natl Acad Sci USA. 1998. 95(26):15603–15607.
18. Glass DJ. Skeletal muscle hypertrophy and atrophy signaling pathways. Int J Biochem Cell Biol. 2005. 37(10):1974–1984.
19. Altun A, Ugur-Altun B. Melatonin: therapeutic and clinical utilization. Int J Clin Pract. 2007. 61(5):835–845.
20. Park S, Lee SK, Park K, Lee Y, Hong Y, Lee S, Jeon JC, Kim JH, Lee SR, Chang KT, Hong Y. Beneficial effects of endogenous and exogenous melatonin on neural reconstruction and functional recovery in an animal model of spinal cord injury. J Pineal Res. 2012. 52(1):107–119.
21. Oner J, Oner H, Sahin Z, Demir R, Ustünel I. Melatonin is as effective as testosterone in the prevention of soleus muscle atrophy induced by castration in rats. Anat Rec (Hoboken). 2008. 291(4):448–455.
22. Lee S, Shin J, Lee M, Lee S, Lee SR, Chang KT, Hong Y. Effects of melatonin on improvement of neurological function in focal cerebral ischemic rats. Reprod Dev Biol. 2011. 35(2):167–174.
23. Lee S, Shin J, Lee M, Hong Y, Lee S, Lee Y, Lkhagvasuren T, Kim DW, Yang YA, Chang KT, Hong Y. The effects of melatonin and/or exercise on ischemic-reperfusion brain damage in focal cerebral ischemic rats. Neural Regen Res. 2012. In press.
24. Schiaffino S, Reggiani C. Fiber types in mammalian skeletal muscles. Physiol Rev. 2011. 91(4):1447–1531.
25. Lin XJ, Mei GP, Liu J, Li YL, Zuo D, Liu SJ, Zhao TB, Lin MT. Therapeutic effects of melatonin on heatstroke-induced multiple organ dysfunction syndrome in rats. J Pineal Res. 2011. 50(4):436–444.
26. Lee S, Lee HK, Shin J, Hong Y, Lee S, Lee S, Suzuki T, Kang T, Hong Y. Effects of controlled photoperiod on body development in growing juvenile rats. Reprod Dev Biol. 2010. 34(2):89–94.
27. Gonciarz M, Gonciarz Z, Bielanski W, Mularczyk A, Konturek PC, Brzozowski T, Konturek SJ. The pilot study of 3-month course of melatonin treatment of patients with nonalcoholic steatohepatitis: effect on plasma levels of liver enzymes, lipids and melatonin. J Physiol Pharmacol. 2010. 61(6):705–710.