Abstract
BACKGROUND/OBJECTIVES
In this study, potential anti-inflammatory effect of enzymatic hydrolysates from Styela clava flesh tissue was assessed via nitric oxide (NO) production in lipopolysaccahride (LPS) induced RAW 264.7 macrophages and in vivo zebrafish model.
MATERIALS/METHODS
We investigated the ability of enzymatic hydrolysates from Styela clava flesh tissue to inhibit LPS-induced expression of pro-inflammatory mediators in RAW 264.7 macrophages, and the molecular mechanism through which this inhibition occurred. In addition, we evaluated anti-inflammatory effect of enzymatic hydrolysates against a LPS-exposed in in vivo zebrafish model.
RESULTS
Among the enzymatic hydrolysates, Protamex-proteolytic hydrolysate exhibited the highest NO inhibitory effect and was fractionated into three ranges of molecular weight by using ultrafiltration (UF) membranes (MWCO 5 kDa and 10 kDa). The above 10 kDa fraction down-regulated LPS-induced expression of inducible nitric oxide synthase (iNOS) and cyclooxygenase-2 (COX-2), thereby reducing production of NO and prostaglandin E2 (PGE2) in LPS-activated RAW 264.7 macrophages. The above 10 kDa fraction suppressed LPS-induced production of pro-inflammatory cytokines, including interleukin (IL)-1β, IL-6, and tumor necrosis factor (TNF)-α. In addition, the above 10 kDa fraction inhibited LPS-induced phosphorylation of extracellular signal-regulated kinases (ERKs), c-Jun N-terminal kinase (JNK), and p38. Furthermore, NO production in live zebrafish induced by LPS was reduced by addition of the above 10 kDa fraction from S. clava enzymatic hydrolysate.
Inflammation plays a pivotal role in various diseases, such as cardiovascular disease, cancer, rheumatoid arthritis, asthma and bronchitis [1,2]. Among inflammation-related processes, macrophages play an important role in inflammatory diseases and secrete pro-inflammatory cytokines including interleukin-1 beta (IL-1β), IL-6 and tumor necrosis factor-alpha (TNF-α) and inflammation mediators including nitric oxide (NO) and prostaglandins [1]. In addition, inflammatory enzymes such as inducible nitric oxide synthase (iNOS) and cyclooxyenase-2 (COX-2) are associated with development of numerous inflammatory diseases [3]. Thus, inhibition of production of inflammatory mediator is the major therapeutic target for treatment of inflammatory diseases.
Zebrafish has become a useful model system for vertebrate development because of its large clutches, small size, transparency, low cost and physiological similarity to mammals [4,5]. In addition, zebrafish has an advantage for in vivo study because of the rapid development and optical transparency during development [4,7]. Recently, zebrafish has emerged as a model system for use in pharmacological studies for human disease such as inflammation, angiogenesis, hypolipidemic and skin aging [5,6,7,8].
Styela clava, used in this study, is an important cultured aquatic species used for food by people in Korea. Several studies on S. clava have pointed out a variety of biological benefits, including antioxidative [9], anticancer [10] hepatoprotective [11] and antihypertensive effects [12,13]. Although the protein content of S. clava flesh tissue is more than 60%, there remains a paucity of data regarding the biological activities of S. clava flesh tissue protein.
Controlled enzymatic hydrolysis of food proteins may also modify and improve and upgrade the nutritional and functional properties of food proteins [14,15]. The molecular weight of the hydrolyzed food protein is a pivotal factors for effective production of protein hydrolysates to use as functional materials [15]. An ultrafiltration (UF) membrane system is used to obtain various molecular weight fractions of hydrolysates [15,16]. The UF system has the major advantages that the molecular weight distribution of hydrolysates can be controlled by appropriate selection of the UF membrane [17].
The current study was conducted in order to investigate the potential effect of enzymatic hydrolysates and molecular weight fractions from S. clava flesh tissue on inflammation through an in vitro study by determining the effect on LPS-induced into macrophages as well as in an in vivo zebrafish model.
Styela clava was collected between March 2010 and May 2011, and kindly donated by Miduduk Corporated Association (Masan, Korea). Commercial food grade proteases including Protamex, Kojizyme, Neutrase, Flavourzyme and Alcalase from Novo Co. (Novozyme Nordisk, Bagasvaerd, Denmark). Dublecco's modified eagle's medium (DMEM), fetal bovine serum (FBS) and phosphate buffer saline (PBS) were purchased from Gibco BRL Co. (Gaithersburg, MD, USA). Dimethyl sulfoxide (DMSO), 2',7'-Dichlorodihydrofluorescein diacetate (DCFH-DA), and 3-[4,5-dimethylthiazol-2-yl]-2,5-diphenyltetrazoliumbromide (MTT) were purchased from Sigma Co. (St. Louis, MO, USA). The other chemicals and reagents used in this study were of experimental grade.
The hydrolytic enzymes used in preparation of enzymatic hydrolysates, eight proteases (Protamex, Kojizyme, Neutrase, Flavourzyme, Alcalase, pepsin, tyrpsin and papain) hydrolyzed the powder of S. clava flesh tissue. The dried ground S. clava flesh tissue powder was homogenized with buffer, and hydrolyzed with enzymes in a substrate to enzyme ratio of 100 : 1 (w/w). The pH of the homogenates was adjusted to its optimal pH value before the enzymatic hydrolysis. The enzymatic reactions were performed for 12 h in order to achieve an optimal degree of enzymatic hydrolysis (Table 1). The hydrolysates were then boiled for 10 min at 100℃ in a water bath to inactivate the enzyme reaction. Each enzymatic hydrolysate was clarified by centrifugation (3500 rpm, for 20 min at 4℃) for removal of the residue. The resultant SFTPH was fractionated through UF membranes with a range of molecular weight (MW) cut-off of 5 and 10 kDa, respectively. Fractionates were designed as follows: SFTPH-I with distribution of above 10 kDa, SFTPH-II with distribution of 5~10 kDa and SFTPH-III with distribution of below 5 kDa. All recovered SFTPH fractions were lyophilized and stored under -20℃ until used.
The murine macrophage cell line RAW 264.7 was purchased from the Korean macrophages Line Bank (KCLB; Seoul, Korea). RAW 264.7 macrophages were grown in DMEM (GIBCO INC., NY, USA) supplemented with 10% heat-inactivated fetal bovine serum (FBS; GIBCO), streptomycin (100 mg/ml), and penicillin (100 unit/ml). Cultures were maintained at 37℃ in 5% CO2 incubator.
RAW 264.7 macrophages (1.0 × 105 cells/ml) plated in a 96-well plate were pre-incubated and then treated with lipopolysaccharide (LPS; 1 µg/ml) plus aliquots of the hydrolysates in sterile distilled water. The cells were then incubated for an additional 24 h at 37℃. MTT stock solution (50 µl; 2 mg/ml in PBS) was then added to each well to a total reaction volume of 250 µl. After incubation for 4 h, the plates were centrifuged (800 × g, 5 min), and the supernatants were aspirated. The formazan crystals in each well were dissolved in 150 µl of dimethylsulfoxide (DMSO), and the absorbance was measured using an ELISA plate reader at 540 nm.
After pre-incubation of RAW 264.7 macrophages (1.0 × 105 cells/ml) with LPS (1 µg/ml) for 24 h, the quantity of nitrite accumulated in the culture medium was measured as an indicator of NO production. In brief, 100 µl of cell culture medium was mixed with 100 µl of Griess reagent [1% sulfanilamide and 0.1% naphthylethylenediamine dihydrochloride in 2.5% phosphoric acid], the mixture was incubated at room temperature for 10 min, and the absorbance at 540 nm was measured in a microplate reader. Fresh culture medium was used as a blank in every experiment.
The three molecular weight fractions (SFTPH-I, SFTPH-II and SFTPH-III) were investigated for NO inhibitory activity. Among the three fractions, the highest NO inhibitory fraction (active fraction) was determined for cytokine inhibitory activities. The active fraction was diluted with DMEM prior to treatment. Cells were treated with LPS (1 µg/ml) for 24 h to allow cytokine production. The PGE2 concentration in the culture medium was quantified using a competitive enzyme immunoassay kit (R&D Systems, Minneapolis, MN, USA) in accordance with the manufacturer's instructions. Production of PGE2 was measured relative to that observed after control treatment.
The active fraction was solubilized with sterile distilled water before treatment. The inhibitory effect of the active fraction on pro-inflammatory cytokine (TNF-α, IL-β and IL-6) production from LPS (1 µg/ml) treated RAW 264.7 macrophages was determined as described by Cho et al. [18]. Supernatants were used for pro-inflammatory cytokine assay using a mouse ELISA kit (R&D Systems, Minneapolis, MN, USA).
The cell lysates were prepared with lysis buffer (20 mM Tris, 5 mM EDTA, 10 mM Na4P2O7, 100 mM NaF, 2 mM Na3VO4, 1% NP-40, 10 mg/ml aprotinin, 10 mg/ml leupeptin and 1 mM PMSF) for 60 min and then centrifuged at 12,000 rpm for 15 min at 4℃. The protein concentrations were determined using a BCA™ protein assay kit. The lysates containing 30 µg of protein were subjected to electrophoresis on sodium dodecyl sulfate (SDS)-polyacrylamide gel, and the gel was transferred onto a nitrocellulose membrane. The membrane was blocked in 5% non fat dry milk in TBST (25 mM Tris-HCl, 137 mM NaCl, 2.65 mM KCl, 0.05% Tween 20, pH 7.4) for 2 h. The primary antibodies were used at a 1 : 1000 dilution. Membranes were incubated with the primary antibodies at 4℃ overnight. The membranes were then washed with TBST, followed by incubation with the secondary antibodies used at 1 : 3000 dilutions. Signals were developed using an ECL western blotting detection kit and exposed to a Western imaging system.
All animal procedures were performed in accordance with the National Institute of Health Guide for the Care and Use of Laboratory Animals and were approved by the Institutional Animal Care and Utilisation Committee for Veterinary Medicine of Jeju National University. Adult zebrafishes were purchased from a commercial dealer (Seoul aquarium, Korea) and 10 fishes were kept in a 3 L acrylic tank with the following conditions; 28.5℃, with a 14/10 h light/dark cycle. Zebrafishes were fed three times a day, 6 d/week, with tetamin flake food supplemented with live brine shrimps (Artemia salina). Embryos were obtained from natural spawning, which was induced in the morning by turning on the light. Collection of embryo was completed within 30 min.
From approximately two days post-fertilization (dpf), embryos (n = 25) were transferred to individual wells of a 24-well plate and maintained in embryo media containing 1 ml of vehicle (sterile distilled water) or 50, 100 and 200 µg/ml of active fraction for 1 h followed by treatment with LPS (3 µg/ml) or co-treatment with active fraction and LPS.
Image analysis of intracellular NO generation was performed according to the method used by Wigesinghe et al. [19]. Generation of NO in an inflammatory zebrafish model was analyzed using a fluorescent probe dye, diaminofluorophore 4-amino-5-methylamino-2-,7-difluorofluorescein diacetate (DAF-FM DA). Transformation of DAF-FM DA by NO in the presence of dioxygen generates highly fluorescent triazole derivatives. The embryos were treated with 50, 100 and 200 µg/ml of active fraction, and, 1 h later, LPS (3 µg/ml) was applied to the plate. Following active fraction and LPS treatment, the zebrafish embryos were transferred into 96-well plates, treated with DAF-FM DA solution (20 µg/ml), and incubated for 1 h in the dark at 28.5℃. After incubation, the zebrafish embryos were rinsed in fresh zebrafish embryo medium and anesthetized in tricaine methanesulfonate solution (1 : 500 dilutions) before observation. Fluorescence intensity of individual zebrafish larvae and embryos was quantified at an excitation wavelength of 485 nm and an emission wavelength of 535 nm, using a Perkin-Elmer LS-5B spectrofluorometer. Images of stained embryos were observed using a florescence microscope equipped with a Moticam color digital camera (Olympus Optical, JP/BX60).
Prior to evaluation of the NO inhibitory effect of enzymatic hydrolysates, we first examined its cytotoxic effect on the viability of RAW 264.7 macrophages. Cytotoxicity of enzymatic hydrolysates was evaluated using the MTT assay at a concentration of 200 µg/ml. None of the enzymatic hydrolysates affected the cytotoxicity of RAW 264.7 macrophages (Fig. 1). Thus, those concentrations were used in subsequent experiments.
To evaluate the question of whether the enzymatic hydrolysates possess a potential anti-inflammatory effect in LPS-stimulated RAW 264.7 macrophages, we investigated inhibitory effect of nine hydrolysates on NO production (Fig. 1). Among the hydrolysates, Protamex hydrolysate exhibited the highest level of inhibitory effect on NO production. Thus, Protamex hydrolysate was selected for further experiments and fractionation of the hydrolysate using UF membranes is described. In this study, using an UF system, Protamex hydrolysate was fractionated into three individual fractions with three molecular weight (MW) groups of SFTPH-I (MW > 10 kDa), SFTPH-II (MW = 5~10 kDa), and SFTPH-III (MW< 5 kDa), using UF membranes (MW cut-off of 5 and 10 kDa. In the NO inhibitory activity assay (Fig. 2), the highest level of inhibitory effect on NO was exhibited by SFTPH-I.
The inhibitory effect of SFTPH-I on PGE2 production in LPS-induced RAW 264.7 macrophages showed a similar pattern to its effect on NO inhibition, in that SFTPH-I inhibited LPS-induced production of PGE2 (Fig. 3A). Although SFTPH-I had an inhibitory effect on PGE2 production, the effect was not as strong as that exhibited in the inhibition of NO production. To elucidate the mechanism involved in the inhibition of NO generation by SFTPH-I in LPS-induced RAW 264.7 macrophages, we further studied the effect of SFTPH-I on iNOS and COX-2 protein expression by Western Blot. In response to LPS expression of iNOS was markedly increased, and SFTPH-I inhibited iNOS and COX-2 protein (Fig. 3B). These results suggested that SFTPH-I inhibited release of NO and PGE2 by reducing expression of iNOS and COX-2.
To determine the effects of SFTPH-I on production of proinflammatory cytokines such as IL-1β, IL-6, and TNF-α, RAW 264.7 macrophages were incubated with SFTPH-I (50, 100 and 200 µg/ml) in the presence or absence of LPS (1 µg/ml) for 24 h, and the cytokine levels were measured by ELISA. Results showed that pretreatment of macrophages with SFTPH-I resulted in considerably reduced production of IL-1β, IL-6, and TNF-α (Fig. 4A-C).
To determine whether SFTPH-I inhibited the production of pro-inflammatory mediators through effects on the MAPK pathway, we evaluated the effect of SFTPH-I on LPS-induced phosphorylation of p38, JNK, and ERK in RAW 264.7 macrophages using western blotting. The levels of phosphorylated ERK, JNK, and p38 MAPK were elevated in macrophages after treatment with LPS only, whereas the levels of total ERK, JNK, and p38 MAPK did not show significant change (Fig. 5). However, compared to cells treated with LPS only, macrophages pretreated with SFTPH-I showed lower LPS-induced activation of these proteins (Fig. 5).
Prior to evaluation of the NO inhibitory effect of SFTH-I, we first examined its toxicity on the survival rate of zebrafish. SFTH-I and LPS did not affect the toxicity of zebrafish (Fig. 6). Fig. 7 shows a typical fluorescence micrograph of the zebrafish. We found that NO produced by LPS exposure in the yolk sac of zebrafish embryos. The negative control, which contained no SFTH-I or LPS, generated a clear image, whereas the positive control, which was treated with LPS, generated a fluorescence image, which suggests that NO production occurred during LPS treatment in the zebrafish. However, in zebrafish treated with SFTH-I prior to LPS treatment, a reduction in the amount of NO was observed.
NO is a pivotal inflammatory mediator synthesized from arginine by nitric oxide synthesis (NOS) [1,20]. Under pathological condition, NO is increased by the iNOS, which subsequently leads to tissue damage [21]. Thus, NO inhibitors are essential for prevention of inflammatory responses. Recent studies have investigated the effect of marine-derived hydrolysates on LPS-stimulated macrophages for treatment of inflammation [22,23]. In the present study, we examined the anti-inflammatory effect of enzymatic hydrolysates from S. clava flesh tissue on LPS-stimulated macrophages and an in vivo zebrafish model.
To obtain the active anti-inflammation hydrolysate, nine proteases were used in hydrolysis of S. clava flesh tissue protein. Protamex-proteolytic hydrolysate showed the highest NO inhibitory activity in macrophages and was selected from further fractionation using UF membrane. Recently, many studies have reported findings that enzymes are capable of producing bioactive properties when incorporated for hydrolysis of food proteins [24,25,26,27]. The molecular weight of the hydrolyzed food protein is a pivotal factor in production of protein hydrolysates with desired biological materials [17]. One of the methods for fractionation of various molecular weights of hydrolysates is to use UF membranes with different pore size [15,17]. Thus, Protamex hydrolysate was fractionated using an UF system into three individual fractions, and then NO inhibitory activity was examined in macrophages. NO inhibitory activity was widely observed in all of the fractions, thus suggesting that many NO inhibitory substances with various molecular weight ranges were contained in the hydrolysate. Among the fractions, the most potent NO inhibition was noted in an over 10 kDa fraction (SFTPH-I). Further study sought to elucidate the pharmacological effects of SFTPH-I on production of pro-inflammatory mediators, and its mechanism of action, in LPS-stimulated RAW 264.7 macrophages. NO is generated from L-arginine by NOS, a three member enzyme family, including iNOS. In addition, COX-2 is an important mediator of inflammation involving NO and PGE2 generation [28]. PGE2 has been implicated as an important mediator in the processes of inflammation [29]. As previously reported, generation of PGE2 is closely related to NO production [1]. Thus, chemopreventive agents that attenuate COX-2-mediated PGE2 production have had a therapeutic effect in many inflammatory diseases [30]. Our results demonstrated that SFTPH-I inhibited NO and PGE2 production in an LPS-stimulated RAW 264.7 macrophage culture system by reducing the expression of iNOS and COX-2.
In addition, SFTPH-I also reduced the LPS-induced production of TNF-α, IL-6, and IL-1β in a dose-dependent manner. TNF-α is a potent activator of macrophages and can stimulate the production or expression of IL-6, IL-1β, PGE2, collagenase, and adhesion molecules. It elicits a number of physiological effects, including septic shock, inflammation, and cytotoxicity [31]. IL-6, a well-known pro-inflammatory cytokine, is regarded as an endogenous mediator of LPS-induced fever [32]. IL-1β is regarded as a pivotal pro-inflammatory cytokine, primarily released by macrophages, and it plays an important role in the pathophysiology of rheumatoid arthritis [33]. Inflammatory stimuli, such as LPS, induce cytokines in the process of macrophage activation, which mediates tissue response in different phases of inflammation [34,35]. Because of their important roles in inflammatory responses, down-regulation of these pro-inflammatory cytokines is of utmost importance during anti-inflammatory therapy. Here, we showed that SFTPH-I inhibited the LPS-induced production of TNF-α, IL-1β, and IL-6.
MAPKs, including ERK, JNK, and p38 kinase play important roles in many biological processes, including inflammation, proliferation, and differentiation [31]. According to a previous report, MAPKs promote iNOS and COX-2 expression in LPS-stimulated macrophages [36]. In addition, phosphorylation of MAPKs promotes generation of pro-inflammatory cytokines in LPS-stimulated macrophages [37]. Thus, the inhibition of MAPKs activation or function is a key mechanism. SFTPH-I attenuated LPS-induced phosphorylation of ERK, p38 and JNK. Taken together, these results suggest the potential role of ERK, p38 and JNK in SFTPH-I-induced suppression of NO, and pro-inflammatory cytokines in activated macrophages.
The number of chemicals that need to be tested in the field of chemical toxicity and drug discovery is steadily increasing. Thus, the need for high-throughput screening methods also arises, where the use of zebrafish embryos was proposed [38] because of their small size and thus suitability for studies in multi-well plates. Not only toxicity screening applications are imaginable but also applications for clarification of mechanisms of toxicity have been studied [39]. In the current study, the potential NO inhibitory effect of SFTH-I was investigated in zebrafish as an alternative animal model system. When zebrafish were treated with SFTH-I prior to LPS treatment, a dramatic decrease in NO production was observed.
Recently, some anti-inflammatory properties have also been reported in certain bioresource proteins, including microalgae, salmon pectoral fin and mussel [40,41,42]. Potent anti-inflammatory peptides can be derived by enzymatic proteolysis of proteins and may act as potential physiological modulators of metabolism during gastrointestinal digestion of nutrients [43,44]. Bioavailability of nutritional supplements generally designates the proportion of the administered substance capable of being absorbed [45]. In terms of bioactive peptides, the bioavailable fractions are mainly short peptides that survive gastrointestinal digestion and absorption [46]. Therefore, further studies are needed for identification of the mechanism of enzymatic hydrolysate from S. clava flesh tissue for the potent anti-inflammatory effect in animal or human.
In conclusion, we evaluated the NO inhibitory effect of the enzymatic hydrolysates of S. clava protein. The Protamex hydrolysate was found to have the highest NO inhibitory effect and was fractionated using the UF membrane system. The above 10 kDa fraction (SFTPH-I) was a potent inhibitor of NO, PGE2, iNOS, COX-2, IL-6, IL-1β, and TNF-α production in LPS-induced RAW 264.7 macrophages. In addition, the levels of phosphorylated MAPKs in LPS-induced RAW 264.7 macrophages were decreased by treatment with SFTPH-I. SFTPH-I also demonstrated NO inhibitory properties against an LPS-stimulated zebrafish model. Based on the results of this study, it appears that this hydrolysate and molecular weight fraction may have a beneficial effect on treatment of inflammatory diseases.
Figures and Tables
Fig. 1
Inhibitory effect of enzymatic hydrolysates from S. clava protein on LPS-induced NO production in RAW 264.7 macrophages (▪) and cell viability (-♦-).
Production of nitric oxide was assayed in the culture medium of macrophages stimulated with LPS (1 µg/ml) for 24 h in the presence of enzymatic hydrolysates (200 µg/ml). Each value indicates the mean ± SD from three independent experiments. *P < 0.05. A, Alcalase; B, Protamex; C, Neutrase; D, Flavourzyme; E, Kojizyme; F, Pepsin; G, Trypsin; H, Papain.
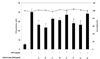
Fig. 2
Inhibitory effects of molecular weight fractions from Protamex hydrolysate on LPS-induced NO production in RAW 264.7 macrophages (▪) and cell viability (-♦-).
Production of nitric oxide was assayed in the culture medium of macrophages stimulated with LPS (1 µg/ml) for 24 h in the presence of molecular weight fractions (50, 100 and 200 µg/ml). Each value indicates the mean ± SD from three independent experiments. *P < 0.05.
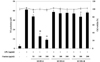
Fig. 3
Effect of SFTPH-I on LPS-induced expression of iNOS and COX-2 and production of PGE2 in RAW 264.7 macrophages. Cells were stimulated with LPS (1 µg/ml) in the presence of SFTPH-I (50, 100, and 200 µg/ml) for 24 h at 37℃.
(A) Culture media were collected for measurement of (A) PGE2 production by ELISA. Values are expressed as mean ± SD of triplicate experiments. *P < 0.05 for comparison with the LPS-stimulated group. (B) Cells were stimulated with LPS (1 µg/ml) in the presence of SFTPH-III (50, 100, and 200 µg/ml) for 24 h at 37℃. The levels of iNOS and COX-2 proteins in cell lysates were analyzed by Western blot.
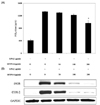
Fig. 4
Inhibitory effect of SFTPH-I on LPS-stimulated IL-6, IL-1β and TNF-α production in RAW 264.7 macrophages.
Production of IL-6, IL-1β and TNF-α was assayed in the culture medium of macrophages stimulated with LPS (1 µg/ml) for 24 h in the presence of SFTPH-I (50, 100 and 200 µg/ml). Levels of IL-6, IL-1β and TNF-α were measured in culture media using an ELISA kit. Each value indicates the mean ± SD from three independent experiments. *P < 0.05, **P < 0.01.
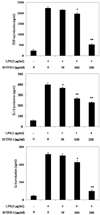
Fig. 5
Inhibitory effect of SFTPH-I on the protein level of p38, ERK and JNK in RAW 264.7 macrophages.
RAW 264.7 macrophages were pre-incubated for 18 h, followed by stimulation with LPS (1 µg/ml) for 20 min in the presence of SFTPH-I (50, 100 and 200 µg/ml). The levels of p-ERK, ERK, p-JNK, JNK, p-p38 and p-38 were determined via Western blotting.
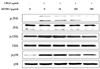
Fig. 6
Survival rate after treatment with LPS or co-treatment with SFTPH-I.
The embryos were treated with LPS (3 µg/ml) and SFTPH-I (50, 100 and 200 µg/ml). Each value indicates the mean ± SD from three independent experiments.
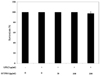
Fig. 7
Inhibitory effect of SFTPH-I on LPS-induced NO production in zebrafish embryos.
The embryos were treated with LPS (3 µg/ml) and SFTPH-I (50, 100 and 200 µg/ml). After incubation, NO was detected by fluorescence after DAF-FM DA staining. The NO level was measured using an image analysis fluorescence microscope. Each value indicates the mean ± SD from three independent experiments. *P < 0.05.
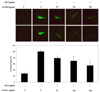
References
1. Heo SJ, Yoon WJ, Kim KN, Ahn GN, Kang SM, Kang DH, Affan A, Oh C, Jung WK, Jeon YJ. Evaluation of anti-inflammatory effect of fucoxanthin isolated from brown algae in lipopolysaccharide-stimulated RAW 264.7 macrophages. Food Chem Toxicol. 2010; 48:2045–2051.


2. Lee SJ, Kim EK, Kim YS, Hwang JW, Lee KH, Choi DK, Kang H, Moon SH, Jeon BT, Park PJ. Purification and characterization of a nitric oxide inhibitory peptide from Ruditapes philippinarum. Food Chem Toxicol. 2012; 50:1660–1666.


3. Kim SY, Jeong HJ, Kim DW, Kim MJ, An JJ, Sohn EJ, Kang HW, Shin MJ, Ahn EH, Kwon SW, Kim DS, Cho SW, Park J, Eum WS, Choi SY. Transduced PEP-1-FK506BP inhibits the inflammatory response in the Raw 264.7 cell and mouse models. Immunobiology. 2011; 216:771–781.


4. Schoonheim PJ, Chatzopoulou A, Schaaf MJ. The zebrafish as an in vivo model system for glucocorticoid resistance. Steroids. 2010; 75:918–925.


5. Ko SC, Cha SH, Heo SJ, Lee SH, Kang SM, Jeon YJ. Protective effect of Ecklonia cava on UVB-induced oxidative stress: in vitro and in vivo zebrafish model. J Appl Phycol. 2011; 23:697–708.


6. Alex D, Lam IK, Lin Z, Lee SM. Indirubin shows anti-angiogenic activity in an in vivo zebrafish model and an in vitro HUVEC model. J Ethnopharmacol. 2010; 131:242–247.


7. Park KH, Cho KH. A zebrafish model for the rapid evaluation of pro-oxidative and inflammatory death by lipopolysaccharide, oxidized low-density lipoproteins, and glycated high-density lipoproteins. Fish Shellfish Immunol. 2011; 31:904–910.


8. Jin S, Cho KH. Water extracts of cinnamon and clove exhibits potent inhibition of protein glycation and anti-atherosclerotic activity in vitro and in vivo hypolipidemic activity in zebrafish. Food Chem Toxicol. 2011; 49:1521–1529.


9. Lee DW, You DH, Yang EK, Jang IC, Bae MS, Jeon YJ, Kim SJ, Lee SC. Antioxidant and ACE inhibitory activities of Styela clava according to harvesting time. J Korean Soc Food Sci Nutr. 2010; 39:331–336.


10. Kim JJ, Kim SJ, Kim SH, Park HR, Lee SC. Antioxidant and anticancer activities of extracts from Styela clava according to the processing methods and solvents. J Korean Soc Food Sci Nutr. 2006; 35:278–283.


11. Kim JM, Park HR, Lee SC, Park E. Ethanol induced leucocytic and hepatic DNA strand breaks are prevented by Styela clava and Styela plicata supplementation in male SD rats. J Korean Soc Food Sci Nutr. 2007; 36:1271–1278.


12. Ko SC, Lee JK, Byun HG, Lee SC, Jeon YJ. Purification and characterization of angiotensin I-converting enzyme inhibitory peptide from enzymatic hydrolysates of Styela clava flesh tissue. Process Biochem. 2012; 47:34–40.


13. Ko SC, Kim DG, Han CH, Lee YJ, Lee JK, Byun HG, Lee SC, Park SJ, Lee DH, Jeon YJ. Nitric oxide-mediated vasorelaxation effects of anti-angiotensin I-converting enzyme (ACE) peptide from Styela clava flesh tissue and its anti-hypertensive effect in spontaneously hypertensive rats. Food Chem. 2012; 134:1141–1145.


14. Chen C, Chi YJ, Zhao MY, Xu W. Influence of degree of hydrolysis on functional properties, antioxidant and ACE inhibitory activities of egg white protein hydrolysate. Food Sci Biotechnol. 2012; 21:27–34.


15. Jeon YJ, Byun HG, Kim SK. Improvement of functional properties of cod frame protein hydrolysates using ultrafiltration membranes. Process Biochem. 1999; 35:471–478.


16. Ko SC, Kang M, Lee JK, Byun HG, Kim SK, Lee SC, Jeon BT, Park PJ, Jung WK, Jeon YJ. Effect of angiotensin I-converting enzyme (ACE) inhibitory peptide purified from enzymatic hydrolysates of Styela plicata. Eur Food Res Technol. 2011; 233:915–922.


17. Byun HG, Kim SK. Purification and characterization of angiotensin I converting enzyme (ACE) inhibitory peptides from Alaska pollack (Theragra chalcogramma) skin. Process Biochem. 2001; 36:1155–1162.


18. Cho JY, Baik KU, Jung JH, Park MH. In vitro anti-inflammatory effects of cynaropicrin, a sesquiterpene lactone, from Saussurea lappa. Eur J Pharmacol. 2000; 398:399–407.


19. Wijesinghe WA, Kim EA, Kang MC, Lee WW, Lee HS, Vairappan CS, Jeon YJ. Assessment of anti-inflammatory effect of 5β-hydroxypalisadin B isolated from red seaweed Laurencia snackeyi in zebrafish embryo in vivo model. Environ Toxicol Pharmacol. 2014; 37:110–117.


20. Samarakoon KW, Ko JY, Shah MM, Lee JH, Kang MC, Kwon ON, Lee JB, Jeon YJ. In vitro studies of anti-inflammatory and anticancer activities of organic solvent extracts from cultured marine microalgae. Algae. 2013; 28:111–119.


21. Kim HK, Cheon BS, Kim YH, Kim SY, Kim HP. Effects of naturally occurring flavonoids on nitric oxide production in the macrophage cell line RAW 264.7 and their structure-activity relationships. Biochem Pharmacol. 1999; 58:759–765.


22. Kim EK, Kim YS, Hwang JW, Kang SH, Choi DK, Lee KH, Lee JS, Moon SH, Jeon BT, Park PJ. Purification of a novel nitric oxide inhibitory peptide derived from enzymatic hydrolysates of Mytilus coruscus. Fish Shellfish Immunol. 2013; 34:1416–1420.


23. Lee SJ, Kim EK, Kim YS, Hwang JW, Lee KH, Choi DK, Kang H, Moon SH, Jeon BT, Park PJ. Purification and characterization of a nitric oxide inhibitory peptide from Ruditapes philippinarum. Food Chem Toxicol. 2012; 50:1660–1666.


24. Hsu KC, Li-Chan EC, Jao CL. Antiproliferative activity of peptides prepared from enzymatic hydrolysates of tuna dark muscle on human breast cancer cell line MCF-7. Food Chem. 2011; 126:617–622.


25. Chen J, Wang Y, Zhong Q, Wu Y, Xia W. Purification and characterization of a novel angiotensin-I converting enzyme (ACE) inhibitory peptide derived from enzymatic hydrolysate of grass carp protein. Peptides. 2012; 33:52–58.


26. Ko SC, Kim D, Jeon YJ. Protective effect of a novel antioxidative peptide purified from a marine Chlorella ellipsoidea protein against free radical-induced oxidative stress. Food Chem Toxicol. 2012; 50:2294–2302.


27. Bougatef A, Nedjar-Arroume N, Manni L, Ravallec R, Barkia A, Guillochon D, Nasri M. Purification and identification of novel antioxidant peptides from enzymatic hydrolysates of sardinelle (Sardinella aurita) by-products proteins. Food Chem. 2010; 118:559–565.


28. Park HY, Han MH, Park C, Jin CY, Kim GY, Choi IW, Kim ND, Nam TJ, Kwon TK, Choi YH. Anti-inflammatory effects of fucoidan through inhibition of NF-κB, MAPK and Akt activation in lipopolysaccharide-induced BV2 microglia cells. Food Chem Toxicol. 2011; 49:1745–1752.


29. Ahmad N, Chen LC, Gordon MA, Laskin JD, Laskin DL. Regulation of cyclooxygenase-2 by nitric oxide in activated hepatic macrophages during acute endotoxemia. J Leukoc Biol. 2002; 71:1005–1011.
30. Höcherl K, Dreher F, Kurtz A, Bucher M. Cyclooxygenase-2 inhibition attenuates lipopolysaccharide-induced cardiovascular failure. Hypertension. 2002; 40:947–953.


31. Kim KN, Heo SJ, Yoon WJ, Kang SM, Ahn G, Yi TH, Jeon YJ. Fucoxanthin inhibits the inflammatory response by suppressing the activation of NF-κB and MAPKs in lipopolysaccharide-induced RAW 264.7 macrophages. Eur J Pharmacol. 2010; 649:369–375.


32. Ghosh S, Karin M. Missing pieces in the NF-kappaB puzzle. Cell. 2002; 109:Suppl. S81–S96.
33. Lee HS, Ryu DS, Lee GS, Lee DS. Anti-inflammatory effects of dichloromethane fraction from Orostachys japonicus in RAW 264.7 cells: suppression of NF-κB activation and MAPK signaling. J Ethnopharmacol. 2012; 140:271–276.


34. Hseu YC, Wu FY, Wu JJ, Chen JY, Chang WH, Lu FJ, Lai YC, Yang HL. Anti-inflammatory potential of Antrodia Camphorata through inhibition of iNOS, COX-2 and cytokines via the NF-kappaB pathway. Int Immunopharmacol. 2005; 5:1914–1925.


35. Laskin DL, Pendino KJ. Macrophages and inflammatory mediators in tissue injury. Annu Rev Pharmacol Toxicol. 1995; 35:655–677.


36. Kyriakis JM, Avruch J. Mammalian MAPK signal transduction pathways activated by stress and inflammation: a 10-year update. Physiol Rev. 2012; 92:689–737.


37. Ajizian SJ, English BK, Meals EA. Specific inhibitors of p38 and extracellular signal-regulated kinase mitogen-activated protein kinase pathways block inducible nitric oxide synthase and tumor necrosis factor accumulation in murine macrophages stimulated with lipopolysaccharide and interferon-gamma. J Infect Dis. 1999; 179:939–944.


38. Hill AJ, Teraoka H, Heideman W, Peterson RE. Zebrafish as a model vertebrate for investigating chemical toxicity. Toxicol Sci. 2005; 86:6–19.


39. Henry TR, Spitsbergen JM, Hornung MW, Abnet CC, Peterson RE. Early life stage toxicity of 2,3,7,8-tetrachlorodibenzo-p-dioxin in zebrafish (Danio rerio). Toxicol Appl Pharmacol. 1997; 142:56–68.


40. Vo TS, Ryu B, Kim SK. Purification of novel anti-inflammatory peptides from enzymatic hydrolysate of the edible microalgal Spirulina maxima. J Funct Foods. 2013; 5:1336–1346.


41. Ahn CB, Cho YS, Je JY. Purification and anti-inflammatory action of tripeptide from salmon pectoral fin byproduct protein hydrolysate. Food Chem. 2015; 168:151–156.


42. Kim EK, Kim YS, Hwang JW, Kang SH, Choi DK, Lee KH, Lee JS, Moon SH, Jeon BT, Park PJ. Purification of a novel nitric oxide inhibitory peptide derived from enzymatic hydrolysates of Mytilus coruscus. Fish Shellfish Immunol. 2013; 34:1416–1420.


43. Byun HG, Lee JK, Park HG, Jeon JK, Kim SK. Antioxidant peptides isolated from the marine rotifer, Brachionus rotundiformis. Process Biochem. 2009; 44:842–846.


44. Je JY, Qian ZJ, Byun HG, Kim SK. Purification and characterization of an antioxidant peptide obtained from tuna backbone protein by enzymatic hydrolysis. Process Biochem. 2007; 42:840–846.

