Abstract
BACKGROUND/OBJECTIVES
This study investigated whether Padina arborescens extract (PAE) protects INS-1 pancreatic β cells against glucotoxicity-induced apoptosis.
MATERIALS/METHODS
Assays, including cell viability, lipid peroxidation, generation of intracellular ROS, NO production, antioxidant enzyme activity and insulin secretion, were conducted. The expressions of Bax, Bcl-2, and caspase-3 proteins in INS-1 cells were evaluated by western blot analysis, and apoptosis/necrosis induced by high glucose was determined by analysis of FITC-Annexin V/PI staining.
RESULTS
Treatment with high concentrations of glucose induced INS-1 cell death, but PAE at concentrations of 25, 50 or 100 µg/ml significantly increased cell viability. The treatment with PAE dose dependently reduced the lipid peroxidation and increased the activities of antioxidant enzymes reduced by 30 mM glucose, while intracellular ROS levels increased under conditions of 30 mM glucose. PAE treatment improved the secretory responsiveness following stimulation with glucose. The results also demonstrated that glucotoxicity-induced apoptosis is associated with modulation of the Bax/Bcl-2 ratio. When INS-1 cells were stained with Annexin V/PI, we found that PAE reduced apoptosis by glucotoxicity.
Glucose, in chronic excess causes toxic effects on the structure and function of organs, including the pancreatic islets [1]. In addition, the role of oxidative stress in the development of diabetes has been emphasized with the presence of reactive oxygen species (ROS) considered as a key factor [2]. Several studies have demonstrated that chronic exposure of β cells to a high glucose concentration resulted in β-cell dysfunction and apoptosis [3]. Dysfunctional and decreasing numbers of pancreatic β cells are determining factors in the development of type 2 diabetes [4]. In addition, β cell death is likely a consequence of intracellular changes caused by chronic hyperglycemia, specifically the increase in mitochondrial oxidative stress and the decrease of reactive oxygen species (ROS)-scavenging enzymes [5]. Therefore, in order to reduce the risk of such pathological damage caused by diabetes, it is important to find ways to attenuate the oxidative stress and apoptosis induced by hyperglycemia.
Although many synthetic compounds that target the regulation of apoptosis in β cells are at different phases of natural products have been investigated that are claimed to have antiapoptotic and antioxidative effects. In particular, marine algae are known to generate an abundance of bioactive compounds with great potential in the pharmaceuticals, food, and biomedical industries [6]. Padina arborescens, a type of brown alga popular in Korea and Japan as a food ingredient and marine herb, contains biologically active compounds such as phlorotannins [7].
Oxidative stress is considered as one of the important mechanisms involved in pancreatic β cell glucose toxicity [1]. In previous studies, we evaluated the antioxidant properties of Padina arborescens extract (PAE) [8]. We also evaluated the effects of PAE on postprandial hyperglycemia by in vivo tests and showed the prominent effect of PAE in both streptozotocin-induced diabetic mice and normal mice [9]. However, there is currently no evidence of a direct involvement of PAE on pancreatic β cell functions and diabetes-related survival. Therefore, in this study, we investigated the protective effects of a PAE against high glucose-induced oxidative stress and apoptosis in INS-1 pancreatic β cells.
The brown alga, Padina arborescens (Phylum Ochrophyta, Class Phaeophyceae, Order Dictyotales, Family Dictyotaceae) was collected along the coast of Jeju Island, Korea. The sample was washed three times with tap water to remove the salt, epiphytes, and sand attached to the surface, then carefully rinsed with fresh water and maintained in a medical refrigerator at -20℃. Thereafter, the frozen samples were lyophilized and homogenized with a grinder prior to extraction. Padina arborescens was extracted with ten volumes of 80% methanol for 12 h at room temperature three times. The filtrate was then evaporated at 40℃ to obtain the methanol extract. The PAE was thoroughly dried for complete removal of solvent and stored in a deep freezer (-80℃).
Rat insulinoma cell line INS-1 was cultured in an RPMI 1640 medium (Gibco, Carlsbad, CA, USA) supplemented with 10% fetal bovine serum (FBS), streptomycin (100 µg/ml), and penicillin (100 units/ml) at 37℃ in a humidified atmosphere containing 5% CO2. The cells were passaged weekly after they had been detached with trypsin-ethylenediaminetetraacetic acid (trypsin-EDTA). All the studies were performed on INS-1 cells that were between passages 20 and 30.
Cell viability was assessed by a colorimetric 3-(4,5-dimethylthiazol-2-yl)-2,5-diphenyltetrazolium bromide (MTT) assay, which is based on the conversion of MTT to MTT-formazan by mitochondrial enzymes, as described previously [10]. Cells (2 × 104 cells/well) in the wells of a 96-well plate were preincubated with glucose (5.5 or 30 mM) for 48 h and then incubated with or without the indicated concentrations of PAE for 48 h. Next, 100 µl of MTT solution (Sigma-Aldrich Co., St. Louis, MO, USA) was added to each well of the 96-well culture plate and incubated for 4 h at 37℃, before the medium containing MTT was removed. The incorporated formazan crystals in the viable cells were rendered soluble with 100 µl of dimethyl sulfoxide (Bio Basic Inc., NY, USA), and the absorbance at 540 nm of each well was read using a microplate reader.
The intracellular ROS level was measured using a dichlorofluorescein assay [11]. 2,7-dichlorodihydrofluorescein diacetate (DCF-DA) can be deacetylated in cells by reacting it quantitatively with intracellular radicals to convert in to its fluorescent product, DCF, which is retained within the cells. Therefore, DCF-DA was used to evaluate the generation of ROS in oxidative damage. Cells (2 × 104 cells/well) were seeded in 96-well black plates and pre-incubated with glucose (5.5 and 30 mM) in a humidified atmosphere containing 5% CO2 at 37℃ for 48 h. After 48 h of incubation, the cells were treated with various concentrations (25, 50, and 100 µg/ml) of PAE and further incubated for 48 h. Thereafter, the medium was removed and the cells were washed twice with phosphate-buffered saline (PBS, pH 7.4) and incubated with 100 µM DCF-DA for 90 min at room temperature. Fluorescence was measured using a fluorescence plate reader (BMG LABTECH GmbH, Offenburg, Germany).
Lipid peroxidation was measured by the production of thiobarbituric acid reactive substances (TBARS). Cells (2 × 104 cells/well) were seeded in 96-well plates and pre-incubated with glucose (5.5 and 30 mM) in a humidified atmosphere containing 5% CO2 at 37℃ for 48 h. After 48 h of incubation, the cells were treated with various concentrations (25, 50, and 100 µg/ml) of PAE and further incubated for 48 h. A 200 µl sample of each medium supernatant was mixed with 400 µl of TBARS solution and then boiled at 95℃ for 20 min. The absorbance at 532 nm was measured and TBARS concentrations were extrapolated from the 1,1,3,3-tetraethoxypropane serial dilution standard curve. Thiobarbituric acid reactive substances values were then expressed as equivalent nanomoles of malondialdehyde (MDA) [12].
The amount of nitrite accumulation, the end-product of NO generation, was assessed by the Griess reaction [13]. Cells (2 × 104 cells/well) were seeded in 96-well plates and pre-incubated with glucose (5.5 and 30 mM) in a humidified atmosphere containing 5% CO2 at 37℃ for 48 h. After 48 h of incubation, the cells were treated with various concentrations (25, 50, and 100 µg/ml) of PAE and further incubated for 48 h. Thereafter, each 50 µl of culture supernatant was mixed with an equal volume of Griess reagent [0.1% N-(1-naphthyl)-ethylenediamine, 1% sulfanilamide in 5% phosphoric acid] and incubated at room temperature for 10 min. The absorbance at 550 nm was measured in a microplate absorbance reader, and a series of known concentrations of sodium nitrite was used as the standard curve.
Cells (2 × 105 cells/dish) in a 10-mm dish were preincubated with glucose (5.5 or 30 mM) for 48 h, and then incubated with or without the indicated concentrations of PAE for 48 h. The medium was removed and the cells were washed twice with PBS. One milliliter of 50 µM potassium phosphate buffer with 1 mM EDTA (pH 7.0) was added, and the cells were scraped off. Each cell suspension was sonicated three times for 5 s on ice each time, and then the sonicated cells were centrifuged at 10,000 g for 20 min at 4℃. The cell supernatant was used to assess the antioxidative enzyme activities. The protein concentration was measured by the method of Bradford, using bovine serum albumin (BSA) as the standard. Superoxide dismutase (SOD) activity was determined by monitoring the auto-oxidation of pyrogallol, one unit of SOD activity being defined as the amount of an enzyme that inhibited the rate of oxidation of pyrogallol [14]. Catalase (CAT) activity was measured according to the method of Aebi [15] by following the decreased absorbance of H2O2 the decrease in the absorbance at 240 nm being measured for 2 min, standards containing 0, 0.2, 0.5, 1 and 2 mM of H2O2 were used to construct the standard curve. Glutathione peroxidase (GSH-px) activity was measured by the method of Lawrence and Burk [16] one unit of GSH-px activity being defined as the amount of enzyme that oxidized 1 nM nicotinamide adenine dinucleotide phosphate (NADPH) consumed per minute.
Cells (2 × 105 cells/well) in 6-well plates were preincubated with glucose (5.5 or 30 mM) for 48 h, and then incubated without or with the indicated concentrations of PAE for 48 h. Thereafter, the medium was carefully removed and the cells were washed with PBS, and replaced with fresh medium containing 3 mM glucose and 2% FBS. After 5 h of incubation, the cells were stimulated with Krebs-Ringer buffer [119 mM NaCl, 4.75 mM KCl, 2.54 mM CaCl2, 1.2 mM MgSO4, 1.2 mM KH2PO4, 5 mM NaHCO3, and 20 mM HEPES, pH 7.4] containing 5 or 25 mM glucose for 60 min at 37℃, and then the medium was collected for detection of insulin secretion using methods previously described [17]. Insulin secretion was determined by Rat/Mouse Insulin enzyme-linked immunosorbent assay (ELISA) kit (Lingo Research, Lingo, MO, USA).
Cells were homogenized with ice-cold lysis buffer containing 250 mM NaCl, 25 mM Tris-HCl (pH 7.5), 1% v/v NP-40, 1 mM dithiothreitol (DTT), 1 mM phenylmethylsulfonyl fluoride (PMSF), and protein inhibitor cocktail (10 µg/ml aprotinin, 1 µg/ml leupeptin). The cells were then centrifuged at 20,000 g for 15 min at 4℃. The supernatants were used as total protein extracts [18]. The total and nuclear protein contents were determined by the Bio-Rad protein kit with BSA as the standard. The lysate containing 20 µg of protein was subjected to electrophoresis on 10% sodium dodecyl sulfate-polyacrylamide gel (SDS-PAGE). Separated proteins were transferred electrophoretically to a pure nitrocellulose membrane, blocked with 5% skimmed milk solution for 1 h, and then incubated with primary antibodies (Abcam, Cambridge, UK; 1:1000) overnight at 4℃. After washing, the blots were incubated with goat anti-rabbit or goat anti-mouse IgG horseradish peroxidase-conjugated (HRP-conjugated) secondary antibody for 1 h at room temperature. Each antigen-antibody complex was visualized using ECL Western Blotting Detection Reagents and detected by chemiluminescence with LAS-1000 plus (FUJIFLIM, Tokyo, Japan). Band densities were determined by an image analyzer (Multi Gauge V3.1, FUJIFILM Corporation, Valhalla, NY, USA) and normalized to β-actin for total protein and nuclear protein.
Cell death by apoptosis was quantified in 5.5 mM and 30 mM glucose with or without PAE-treated cells by Annexin V-fluorescein isothiocyanate (FITC)/propidium iodide (PI) double staining. Annexin V-FITC/PI double staining of the cells was measured using the Annexin V-FITC kit (Merck bioscience, Darmstadt, Germany). Thereafter, 100 µl of the cell suspension was incubated with 5 µl of Annexin V and 5 µl of PI. The cells were kept in the darkness for 15 min in an ice bath, before 400 µl of a binding buffer was added to the cell suspension, and flow cytometry was promptly read by FACScan flow cytometer. Cells in the Annexin V-FITC-/PI-population were regarded as normal healthy cells, while Annexin V-FITC+/PI-status was considered as the measure of early apoptosis, Annexin V-FITC+/PI+ as late apoptosis, and Annexin V-FITC-/PI+ as necrosis. The apoptotic cells are expressed as a percentage of the total number of cells.
Each experiment was performed in triplicate. The results were expressed as the mean ± SD. Statistical analysis, including analysis of variance, was performed using SAS 9.1 software. Significant differences (P < 0.05) between the means were determined by Duncan's multiple range tests.
The effect of PAE on cell viability in INS-1 pancreatic β cells was examined using the MTT assay. When INS-1 cells were treated with 30 mM glucose for 48 h, cell viability was reduced significantly. As shown in Fig. 1, the viability of INS-1 cells exposed to PAE was significantly increased compared with 30 mM glucose treatment. In particular, treatment with 100 µg/ml of PAE along with high glucose treatment resulted in a significant increase in cell viability, to 83.03%.
High glucose has been shown to increase ROS in many cell types in patients with diabetes owing to a combination of increased production of ROS along with decreased antioxidant function [19,20]. As shown in Fig. 2, the generation of intracellular ROS in INS-1 cells was elevated significantly after treatment with 30 mM glucose as compared with that in cell treated with 5.5 mM normal glucose. However, PAE treatment dose-dependently reduced the levels of ROS in the cells induced by treatment with 30 mM.
Fig. 3 shows the effect of PAE on lipid peroxidation in the high glucose treated INS-1 cells by measuring TBARS, a lipid peroxidation. The TBARS level of the normal glucose treated cells (5.5 mM glucose) was recorded as 0.12 nmol MDA, whereas that of the high glucose-treated cells was recorded as 0.27 nmol MDA. However, treatment with PAE together with high glucose significantly inhibited TBARS formation, indicating protection against lipid peroxidation.
Fig. 4 shows that the level of NO in INS-1 cells was significantly increased by the 30 mM glucose treatment as compared with the cells treated with 5.5 mM normal glucose. However, the high glucose-induced NO level was significantly suppressed by treatment with PAE. The level of NO in the INS-1 cells treated with 30 mM glucose was 204.38%, but treatment with 100 µg/ml of PAE in conjunction with high glucose exposure resulted in a significantly lower NO level of 88.8%. Thus, the PAE was able to prevent NO production stimulated by high glucose.
Overexpression of antioxidant enzymes (i.e. SOD, CAT, GSH-px) in β cell lines provide protection against pro-oxidants [21]. The effects of PAE on antioxidant enzyme activities in high glucose-treated INS-1 cells are shown in Table 1. The 30 mM glucose treatment reduced SOD activity compared with that measured in the cells treated with 5.5 mM normal glucose. Treating the 30 mM glucose-treated INS-1 cells with PAE increased the SOD activity, as shown by a SOD activity of 39.04 unit/mg of protein at a dosage of 100 µg/ml. In addition, treatment for 48 h with 30 mM glucose significantly attenuated the CAT activity of INS-1 cells compared that seen in the cells pretreated with 5.5 mM glucose. PAE treatment increased CAT activity in a dose-dependent manner. In addition, GSH-px activity in INS-1 cells treated with high glucose was reduced significantly in comparison to the 5.5 mM normal glucose. After the cells were treated with 100 µg/ml of PAE, GSH-px activity was increased significantly, to 2.32 unit/mg of protein.
As shown in Fig. 5, glucose-stimulated insulin secretion in INS-1 cells was reduced significantly as the result of 30 mM glucose treatment as compared with 5.5 mM normal glucose treatment. In addition, after culturing with high glucose, stimulation with 25 mM glucose resulted in a 2-fold increase in insulin secretion as compared with 5 mM glucose treatment. Treatment with PAE increased insulin secretion in the cells stimulated with 5 and 25 mM glucose under high glucose conditions.
To determine whether PAE induces expression of proteins related to high glucose-induced apoptosis, PAE concentrations of 50 and 100 µg/ml were added to INS-1 cells (Fig. 6). Exposure to high glucose resulted in a decrease in Bcl-2 anti-apoptotic protein expression, whereas expression of Bax pro-apoptotic protein increased, thereby increasing Bax/Bcl-2 ratios. However, the cells treated with PAE showed lower Bax expression than the 30 mM glucose-treated cells. Furthermore, the Bcl-2 expression level following treatment with PAE was markedly increased. The pro-apoptotic index, the caspase-3 expression, was augmented by high glucose concentration, and this was significantly reduced by PAE.
Fig. 7 shows the results of a flow cytometric analysis with Annexin V/PI double staining. When INS-1 cells were treated with 30 mM glucose, the level of apoptosis was higher than that in the cells treated with 5.5 mM normal glucose. However, flow cytometric analysis demonstrated that treatment of cells with PAE increased the number of normal cells (Annexin V-FITC-/PI-) in a dose-dependent manner. The apoptotic cells including early apoptotic (Annexin V-FITC+/PI-) and late apoptotic cells (Annexin V-FITC+/PI+) that were reduced in a dose-dependent manner.
In this study, we investigated the anti-apoptotic effects of Padina arborescens extract (PAE) using high glucose treated INS-1 pancreatic β cells. In using this diabetic experimental cell model, we have determined that PAE derives its anti-apoptotic properties through reducing oxidative stress. Treatment with high glucose concentrations for a long period inflicts glucotoxicity on vulnerable cell types such as pancreatic β cells. Glucotoxicity is thought to play a major role, at least in significant part by increasing oxidative stress, in the apoptosis of pancreatic β cells [1,20]. In addition, oxidative stress leads to cellular damage, which plays a central role in the development of β cell dysfunction, insulin resistance, and diabetic complications [21,22]. Pancreatic β cell dysfunction plays a key role in the pathogenesis of type 2 diabetes. Therefore, in order to reduce the risk of such pathological damage, it is important to find ways to attenuate the oxidative stress and apoptosis induced by glucotoxicity.
Recently, there has been some focus on the toxic effects of fluctuating high glucose on islet β cell functions and survival [23]. In this study, the exposure of INS-1 cells to a high level of glucose resulted in significantly reduced cell viability (Fig. 1). However, PAE treatment was shown to inhibit cell death. Therefore, this study demonstrated that PAE protects INS-1 cells against high-glucose-induced cytotoxicity.
On the other hand, the evidence for accumulation of ROS leading to β cell dysfunction and apoptosis has been reviewed recently [24]. Our results demonstrated that the treatment of INS-1 cells with 30 mM glucose significantly increased intracellular ROS levels. However, PAE inhibited high glucose-induced ROS production (Fig. 2). Excessive ROS production causes damage to cellular components; RNA is especially vulnerable, potentially leading to gene expression changes [25]. Therefore, our results revealed that PAE could prevent high glucose-induced pancreatic β cell damage through the reduction of ROS generation.
ROS are also known to cause lipid peroxidation and reactive lipid aldehyde formation, which contribute to progressive deterioration of β cell function in type 2 diabetes. Moreover, the lipid peroxidation can lead to cell apoptosis, and it is considered to be implicated in a number of pathophysiological conditions [26]. In this study, a high glucose treatment induced lipid peroxidation in INS-1 cells, and PAE was found to effectively inhibit TBARS formation (Fig. 3). The protective action of PAE on TBARS formation can be attributed to its antiperoxidative effects.
High glucose treatment leads to overproduction of NO [27]. Nitric oxide induces cell death through the activation of the intrinsic apoptotic pathway [28]. In addition, NO production appears to be involved in β cell damage, dysfunction, and death and the pathogenesis of diabetes [29]. As shown in Fig. 4, the level of NO in INS-1 cells was significantly elevated as the result of 30 mM glucose treatment as compared with the cells treated with 5.5 mM normal glucose. However, NO levels in the PAE-treated cells were reduced significantly, and this effect was concentration dependent. These findings suggest that PAE might confer important protection against the β cell damage induced by hyperglycemia.
The link between oxidative stress and glucotoxicity has been suggested in earlier studies on β cell lines, isolated islets, and diabetic animal models showing that antioxidants can protect β cells against the deleterious effects of high glucose levels on insulin secretion, insulin gene expression, islet insulin content and survival [30,31]. In the present study we observed that the application of a high-glucose pretreatment to INS-1 cells with PAE resulted in increased antioxidant enzyme activities (Table 1). Considering these results, PAE may increase the level of antioxidative enzymes in INS-1 cells. In pancreatic β cells, Grankvist et al. demonstrated that treatment with SOD prevented alloxan-induced diabetes in mice [32]. Catalase overexpression protects islets against oxidative stress [33]. Furthermore, GSH-px seems to be the most important tool to protect the cells against oxidative damage [34]. As pancreatic β cells express a low level of antioxidants [35], the increased ROS production probably results in an oxidative stress that may affect the survival of β cells. Taken together, these effects are mediated by reducing the oxidative stress, being associated with reduced ROS generation and increased cell survival. In addition, as shown in Fig. 5, PAE treatment improved the secretory responsiveness following stimulation with glucose. In this study, the results demonstrate that PAE increases insulin secretion in β cells under high glucose conditions and the mechanisms may be involved in the protection of insulin-secreting cells by reducing oxidative stress and increasing cell function.
Toxic oxidative stress is also an important reason for pancreatic β cell apoptosis [36]. The imbalance of expression of anti- and proapoptotic protein after the stimulus is one of the major mechanisms underlying the ultimate fate of cells in the apoptotic process. It is well-known that the proteins of the Bcl-2 family play a pivotal role in cells undergoing apoptosis by interfering with the caspases [37]. In addition, caspase-3 is a member of the caspase family that has been shown to play an essential role in apoptosis induced by a variety of stimuli [38]. In this study, PAE can modulate the process of apoptosis in INS-1 cells through caspase-3 decrease, an increase of Bcl-2 expression, and a reduction in Bax expression (Fig. 6). These effects were mediated by suppressing apoptosis and were associated with an increase in anti-apoptotic Bcl-2 expression and a reduction pro-apoptotic Bax and caspase-3 expression. In addition, the exposure of INS-1 cells to high levels of glucose resulted in increases in cell apoptosis rate (Fig. 7). It has been indicated that activation of the apoptosis pathway, which is mediated by oxidative stress, is involved in the progression of β cell death. However, on treating high glucose-stressed INS-1 cells with PAE, the apoptotic population was significantly decreased. The treatment with PAE was found to be most effective in ameliorating mitochondria-mediated apoptosis caused by high glucose stress in INS-1 cells.
To conclude, pancreatic INS-1 β cell damage occurred because of high glucose-induced overproduction of ROS, lipid peroxidation and NO production. High glucose treatments reduced antioxidant enzyme activities and the increased apoptosis in INS-1 cells, while PAE protects cell damage in INS-1 cells under high glucose conditions by reducing oxidative stress and apoptosis. These findings demonstrate that PAE might be employed as a potential nutraceutical agent to protect against the glucotoxicity caused by high glucose-induced oxidative stress and apoptosis.
Figures and Tables
Fig. 1
Effect of PAE on the viability of high glucose treated INS-1 pancreatic β cells. Cells in wells of 96-well plates (2 × 104 cells/well) were preincubated with 5.5 mM or 30 mM glucose for 48 h, and subsequently incubated for 48 h in the presence or absence of 25, 50, or 100 µg/mL PAE. The use of 5.5 mM glucose was representative of normal glucose conditions and the 30 mM glucose treatments represent high glucose conditions. Each value is expressed as mean ± SD (n = 3). a-dValues with different alphabets differ significantly at P < 0.05 as analyzed via Duncan's multiple range test.
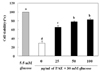
Fig. 2
Effect of PAE on intracellular ROS generation in the high glucose treated INS-1 pancreatic β cells. Cells in wells of 96-well plates (2 × 104 cells/well) were preincubated with glucose and incubated in the absence or presence of PAE as described in the legend to Fig. 1. Each value is expressed as mean ± SD (n = 3). a-dValues with different alphabets differ significantly at P < 0.05 as analyzed via Duncan's multiple range test.
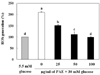
Fig. 3
Effect of PAE on TBRAS generation in the high glucose treated INS-1 pancreatic β cells. Cells in wells of 96-well plates (2 × 104 cells/well) were preincubated with glucose and incubated in the absence or presence of PAE as described in the legend to Fig. 1. Each value is expressed as mean ± SD (n = 3). a-dValues with different alphabets differ significantly at P < 0.05 as analyzed via Duncan's multiple range test.
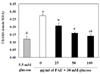
Fig. 4
Effect of PAE on NO level in high glucose treated INS-1 pancreatic β cells. Cells in wells of 96-well plates (2 × 104 cells/well) were preincubated with glucose and incubated in the absence or presence of PAE as described in the legend to Fig. 1. Each value is expressed as mean ± SD (n = 3). a-cValues with different alphabets differ significantly at P < 0.05 as analyzed via Duncan's multiple range test.
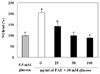
Fig. 5
Effects of PAE on insulin secretion in high glucose-treated INS-1 pancreatic β cells. Cells in wells of 6-well plates (2 × 105 cells/well) were preincubated with glucose and incubated in the absence or presence of PAE as described in the legend to Fig. 1. Each value is expressed as mean ± SD (n = 3). a-dValues with different alphabets differ significantly at P < 0.05 as analyzed via Duncan's multiple range test.
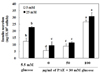
Fig. 6
Effects of PAE on the expression of Caspase-3, Bax and Bcl-2 (A) and Caspase-3, Bax and Bcl-2 ratio (B) in INS-1 pancreatic β cells. Relative expression was quantified by densitometry using the Multi Gauge V3.1 and calculated according to the reference bands of β-actin (mean, n = 3). a-cValues with different alphabets differ significantly at P < 0.05 as analyzed via Duncan's multiple range test.
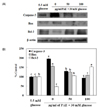
Fig. 7
Identification of the type of cell death by Annexin V-FITC/PI staining. The status of cell death was determined by counting the cells stained with Annexin V-FITC/PI using glow cytometer. Cells were preincubated with glucose and incubated in the absence or presence of PAE as described in the legend to Fig. 1. A: 5.5 mM glucose; B: 30 mM glucose; C: 30 mM glucose + PAE 50 µg/mL; D: 30 mM glucose + PAE 100 µg/mL.
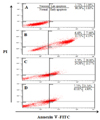
Table 1
Effects of PAE on antioxidative enzyme activities in high glucose treated INS-1 pancreatic β cells

Cells in wells of 10mm dishes (1 × 106 cells/dish) were preincubated with glucose and incubated in the absence or presence of PAE as described in the legend to Fig. 1. Each value is expressed as mean ± SD (n = 3). a-dValues with different alphabets differ significantly at P < 0.05 as analyzed via Duncan's multiple range test. SOD: Super oxide dismutase activity; CAT: Catalase activity; GSH-px: Glutathione peroxidase activity.
References
1. Robertson RP. Chronic oxidative stress as a central mechanism for glucose toxicity in pancreatic islet beta cells in diabetes. J Biol Chem. 2004; 279:42351–42354.


2. Zúrová-Nedelcevová J, Navarová J, Drábiková K, Jancinová V, Petríková M, Bernátová I, Kristová V, Snirc V, Nosál'ová V, Sotníková R. Participation of reactive oxygen species in diabetes-induced endothelial dysfunction. Neuro Endocrinol Lett. 2006; 27:Suppl 2. 168–171.
3. Mandrup-Poulsen T, Helqvist S, Wogensen LD, Mølvig J, Pociot F, Johannesen J, Nerup J. Cytokine and free radicals as effector molecules in the destruction of pancreatic beta cells. Curr Top Microbiol Immunol. 1990; 164:169–193.
4. Butler AE, Janson J, Bonner-Weir S, Ritzel R, Rizza RA, Butler PC. β-cell deficit and increased β-cell apoptosis in humans with type 2 diabetes. Diabetes. 2003; 52:102–110.


5. Brownlee M. The pathobiology of diabetic complications: a unifying mechanism. Diabetes. 2005; 54:1615–1625.
6. Heo SJ, Park EJ, Lee KW, Jeon YJ. Antioxidant activities of enzymatic extracts from brown seaweeds. Bioresour Technol. 2005; 96:1613–1623.


7. Pal Singh I, Bharate SB. Phloroglucinol compounds of natural origin. Nat Prod Rep. 2006; 23:558–591.


8. Park MH, Han JS. Protective effect of Padina arborescens extract against high glucose-induced oxidative damage in human umbilical vein endothelial cells. Prev Nutr Food Sci. 2013; 18:11–17.


9. Park MH, Han JS. Hypoglycemic effect of Padina arborescens extract in streptozotocin-induced diabetic mice. Prev Nutr Food Sci. 2012; 17:239–244.


10. Fautz R, Husein B, Hechenberger C. Application of the neutral red assay (NR assay) to monolayer cultures of primary hepatocytes: rapid colorimetric viability determination for the unscheduled DNA synthesis test (UDS). Mutat Res. 1991; 253:173–179.


11. Wang H, Joseph JA. Quantifying cellular oxidative stress by dichlorofluorescein assay using microplate reader. Free Radic Biol Med. 1999; 27:612–616.


12. Fraga CG, Leibovitz BE, Tappel AL. Lipid peroxidation measured as thiobarbituric acid-reactive substances in tissue slices: characterization and comparison with homogenates and microsomes. Free Radic Biol Med. 1988; 4:155–161.


13. Nath J, Powledge A. Modulation of human neutrophil inflammatory responses by nitric oxide: studies in unprimed and LPS-primed cells. J Leukoc Biol. 1997; 62:805–816.


14. Marklund S, Marklund G. Involvement of the superoxide anion radical in the autoxidation of pyrogallol and a convenient assay for superoxide dismutase. Eur J Biochem. 1974; 47:469–474.


15. Aebi H. Catalase in vitro. Methods Enzymol. 1984; 105:121–126.
16. Lawrence RA, Burk RF. Glutathione peroxidase activity in selenium-deficient rat liver. Biochem Biophys Res Commun. 1976; 71:952–958.


17. Green CD, Jump DB, Olson LK. Elevated insulin secretion from liver X receptor-activated pancreatic β-cells involves increased de novo lipid synthesis and triacylglyceride turnover. Endocrinology. 2009; 150:2637–2645.


18. Yamabe N, Kang KS, Goto E, Tanaka T, Yokozawa T. Beneficial effect of Corni Fructus, a constituent of Hachimi-jio-gan, on advanced glycation end-product-mediated renal injury in streptozotocin-treated diabetic rats. Biol Pharm Bull. 2007; 30:520–526.


19. Lenzen S. Oxidative stress: the vulnerable beta-cell. Biochem Soc Trans. 2008; 36:343–347.
20. Robertson RP, Harmon J, Tran PO, Poitout V. Beta-cell glucose toxicity, lipotoxicity, and chronic oxidative stress in type 2 diabetes. Diabetes. 2004; 53:Suppl 1. S119–S124.
21. Tiedge M, Lortz S, Munday R, Lenzen S. Complementary action of antioxidant enzymes in the protection of bioengineered insulin-producing RINm5F cells against the toxicity of reactive oxygen species. Diabetes. 1998; 47:1578–1585.


22. Evans JL, Goldfine ID, Maddux BA, Grodsky GM. Oxidative stress and stress-activated signaling pathways: a unifying hypothesis of type 2 diabetes. Endocr Rev. 2002; 23:599–622.


23. Del Guerra S, Grupillo M, Masini M, Lupi R, Bugliani M, Torri S, Boggi U, Del Chiaro M, Vistoli F, Mosca F, Del Prato S, Marchetti P. Gliclazide protects human islet beta-cells from apoptosis induced by intermittent high glucose. Diabetes Metab Res Rev. 2007; 23:234–238.


24. Robertson R, Zhou H, Zhang T, Harmon JS. Chronic oxidative stress as a mechanism for glucose toxicity of the beta cell in type 2 diabetes. Cell Biochem Biophys. 2007; 48:139–146.


25. Kong Q, Lin CL. Oxidative damage to RNA: mechanisms, consequences, and diseases. Cell Mol Life Sci. 2010; 67:1817–1829.


27. Du X, Stocklauser-Färber K, Rösen P. Generation of reactive oxygen intermediates, activation of NF-kappaB, and induction of apoptosis in human endothelial cells by glucose: role of nitric oxide synthase? Free Radic Biol Med. 1999; 27:752–763.


28. Messmer UK, Reed UK, Brüne B. Bcl-2 protects macrophages from nitric oxide-induced apoptosis. J Biol Chem. 1996; 271:20192–20197.


29. McDaniel ML, Kwon G, Hill JR, Marshall CA, Corbett JA. Cytokines and nitric oxide in islet inflammation and diabetes. Proc Soc Exp Biol Med. 1996; 211:24–32.


30. Kaneto H, Fujii J, Myint T, Miyazawa N, Islam KN, Kawasaki Y, Suzuki K, Nakamura M, Tatsumi H, Yamasaki Y, Taniguchi N. Reducing sugars trigger oxidative modification and apoptosis in pancreatic beta-cells by provoking oxidative stress through the glycation reaction. Biochem J. 1996; 320:855–863.


31. Tajiri Y, Grill V. Aminoguanidine exerts a beta-cell function-preserving effect in high glucose-cultured beta-cells (INS-1). Int J Exp Diabetes Res. 2000; 1:111–119.


32. Grankvist K, Marklund S, Täljedal IB. Superoxide dismutase is a prophylactic against alloxan diabetes. Nature. 1981; 294:158–160.


33. Benhamou PY, Moriscot C, Richard MJ, Beatrix O, Badet L, Pattou F, Kerr-Conte J, Chroboczek J, Lemarchand P, Halimi S. Adenovirus-mediated catalase gene transfer reduces oxidant stress in human, porcine and rat pancreatic islets. Diabetologia. 1998; 41:1093–1100.


34. Krause MS, McClenaghan NH, Flatt PR, de Bittencourt PI, Murphy C, Newsholme P. L-arginine is essential for pancreatic beta-cell functional integrity, metabolism and defense from inflammatory challenge. J Endocrinol. 2011; 211:87–97.


35. Moriscot C, Pattou F, Kerr-Conte J, Richard MJ, Lemarchand P, Benhamou PY. Contribution of adenoviral-mediated superoxide dismutase gene transfer to the reduction in nitric oxide-induced cytotoxicity on human islets and INS-1 insulin-secreting cells. Diabetologia. 2000; 43:625–631.

