Abstract
Inorganic arsenic (iAs) is a toxic metalloid found ubiquitously in the environment. In humans, exposure to iAs can result in toxicity and cause toxicological manifestations. Arsenic trioxide (As2O3) has been used in the treatment for acute promyelocytic leukemia. The kidney is the critical target organ of trivalent inorganic As (iAsIII) toxicity. We examine if oral administration of astaxanthin (AST) has protective effects on nephrotoxicity and oxidative stress induced by As2O3 exposure (via intraperitoneal injection) in rats. Markers of renal function, histopathological changes, Na+-K+ ATPase, sulfydryl, oxidative stress, and As accumulation in kidneys were evaluated as indicators of As2O3 exposure. AST showed a significant protective effect against As2O3-induced nephrotoxicity. These results suggest that the mechanisms of action, by which AST reduces nephrotoxicity, may include antioxidant protection against oxidative injury and reduction of As accumulation. These findings might be of therapeutic benefit in humans or animals suffering from exposure to iAsIII from natural sources or cancer therapy.
Astaxanthin (AST) is a carotenoid pigment that occurs naturally in red-orange pigments of seafoods, such as salmon, shells of crabs and shrimps, as well as a wide variety of plants and algae. AST can be obtained through dietary intake of salmon and trout, or from health foods. Humans have consumed these foods since ancient times, and AST is now available as an oral supplement.
AST has potential clinical applications due to its anti-inflammatory, immunomodulatory, and anti-cancer effects via scavenging oxygen radicals, anti-diabetic activities, and powerful antioxidant activity [1]. AST has been reported to exert a neuroprotective effect on damage to neuronal cells by crossing the brain-blood barrier [2]. Topical AST is effective in protecting the ocular surface against ultraviolet exposure without causing adverse effects [3].
Inorganic arsenic (iAs) is a toxic metalloid present ubiquitously in soil and water throughout the world, as well as in foods [4] (particularly seafood) [5]. The toxicity of iAs is largely dependent upon its chemical environment. The inorganic forms of As are the most toxic, followed by monomethylarsonic acid (MMA) and dimethylarsinic acid (DMA); whereas the organic forms of As, in general, are mostly considered to be non-toxic [6]. Therefore, the potential toxicity of iAs is dependent not only on the total As concentration, but also on the species of As present in pollutant sources.
Exposure to iAs can cause serious negative health effects in humans, such as carcinogenesis in the kidney, lungs, and urinary bladder [7]. Poor nutritional status is thought to confer greater susceptibility to As toxicity. The mechanisms underlying iAs-induced diseases and cancers are not precisely understood. However, iAs bound to proteins in organs/tissues and iAs-induced oxidative stress are considered to be the main contributors to iAs-induced carcinogenesis [8]. Recently, oxidative stress has been considered to be one of the major toxic mechanisms [9,10]. The potential role of oxidative stress in injuries associated with iAs poisoning suggests that antioxidants may enhance the efficacy of treatment protocols designed to mitigate iAs-induced toxicity [11,12].
Recent studies have suggested that AST exhibits about 100-500-times higher antioxidant activity than α-Tocopherol [13]. It has been shown that AST is much more effective than vitamin E in protecting the mitochondria in rat liver cells against lipid peroxidation [14]. There are increasing numbers of studies focusing on natural compounds and their possible benefits with regard to human health [15]. However, reports on the effects of AST on As2O3 toxicity are lacking.
In general, trivalent iAs (iAsIII) is more toxic than pentavalent iAs. As trioxide (As2O3) is the most commonly produced form of iAsIII. As2O3 has been employed in the treatment of acute promyelocytic leukemia [16-18], but is a poison. The kidney is the critical target organ of iAsIII toxicity.
In the present study, we examined if oral administration of AST has protective effects on the nephrotoxicity and oxidative stress induced by exposure to As2O3 in Wistar rats.
As2O3 was purchased from Harbin Yida Pharmaceutical Co. Ltd. (Harbin, China). AST was purchased from Dr. Ehrenstorfer GmbH (Augsburg, Germany). All other chemicals used were of analytical grade. The tested dose of As2O3 (5 mg/kg body weight (BW), via intraperitoneal injection) was chosen based on the previous reports [19, 20]. The oral doses of AST (10, 20, and 40 mg/kg BW) were used in the present study according to the studies of Augusti et al. [21], Curek et al. [22], and Lee and Lee [23].
Male 8-week-old Wistar rats from the Experimental Animal Centre of Harbin Veterinary Research Institute of Chinese Academy of Agricultural Sciences (Harbin, China) were housed in the Animal Quarters of the Northeast Agricultural University at 22℃ on a 12-h light-dark cycle, with a mean weight of 150 g. They were allowed free access to standard rodent chow and tap water. Forty rats were assigned randomly to five groups of eight: the control, As2O3-treated (5 mg/kg BW), and three As2O3+AST-treated groups (AST: 10, 20 and 40 mg/kg BW; As2O3: 5 mg/kg BW). As2O3 (via intraperitoneal injection) and AST (oral administration) treatments were given for 5 days with measurements made on the sixth day. An equal amount of 0.9% normal saline was administered as vehicles to the control rats. On the sixth day, the rats were given ether anesthesia and killed. The study protocol was approved (Number 2012020) by the Ethics Committee of Northeast Agricultural University (Harbin, China).
Rats were placed in metabolic chambers to obtain a 24-h urine collection using ice-jacketed 50-mL tubes before the induction of anesthesia. The collected urine samples were allowed to settle for 30 min to remove debris. Then, the urine was aliquoted for various assays. Blood samples were collected into evacuated tubes containing liquaemin acid solution (anticoagulant), which were then centrifuged at 3,000 × g for 10 min. Serum was collected and stored at -20℃ for future analyses. Renal tissues were homogenized in phosphate-buffered saline (PBS) at pH 7.4 using an Ultrathurax T25 Homogenisator (Janke & Kunkel KIKA-Labortechnik, Köln, Germany). The mixture was centrifuged at 10,000 × g for 10 min at 4℃, and the supernatant was collected and stored at -20℃.
As contents in the kidney tissues of all rats were analyzed, following the method of Cui et al. [24] with an atomic fluorescence spectrometry system (AFS930; Beijing Jitian Instrument Co. Ltd., Beijing, China).
Levels of blood urea nitrogen (BUN), creatinine (CREA), lactate dehydrogenase (LDH), alkaline phosphatase (ALP), total albumin (TP), and β-N-acetyl-D-glucosaminidase (NAG) in urine were detected using UniCel DxC800 Synchron chemistry system (Beckman Coulter, Fullerton, CA, USA) with corresponding test kits (Jiancheng Bio-engineering Institute, Nanjing, China).
GSH in the supernatants of samples of renal tissues was determined by the method described by Lakritz et al. [25] and calculated as the ratio of GSH to GSSG.
The amount of ROS in the kidneys was measured using 2',7'-Dichlorofluorescin diacetate, which is converted into highly fluorescent dichlorofluorescin by cellular peroxides (including hydrogen peroxide). The assay was carried out as described previously [26,27]. Fluorescence was determined at an excitation wavelength of 488 nm and emission wavelength of 525 nm using Fluorescence Plate Reader (LS-55, Perkin-Elmer, Buckinghamshire, UK).
The levels of Na+-K+ ATPase and sulfydryl in renal tissues were detected using relevant kits (Jiancheng Bio-engineering Institute, Nanjing, China).
The activities of SOD and CAT, as well as malonaldehyde (MDA) levels in renal tissue, were detected using relevant kits (Jiancheng Bio-engineering Institute, Nanjing, China).
The levels of 8-hydroxy-2'-deoxyguanosine (8-OHdG), which is a marker of oxidative DNA damage, were detected. The DNA of each sample was extracted using DNeasy Tissue kit (Tiangen, Beijing, China). The 8-OHdG levels were measured using oxidative DNA Damage Enzyme-linked Immunosorbent Assay kit (Cell Biolaboratories, San Diego, CA, USA) in accordance to manufacturer's instructions.
The renal tissues were removed rapidly. For light microscopic (BX-FM; Olympus, Tokyo, Japan) observation, renal tissues were fixed by immersion in 4% formaldehyde solution for 24 h, and paraffin sections (4 µm) were cut and stained with hematoxylin & eosin (H&E).
Exposure to As2O3 alone caused a significant (P < 0.01) increase in the serum levels of CREA and BUN in rats, and AST had a dose-dependent protective effect on this increase (Table 1). Increases in the urinary levels of LDH and ALP, as well as NAG activity and TP concentration, were observed in the As2O3 group compared with the control group. These increases correlated negatively with an increase in the dose upon co-administration of AST.
The activities of SOD (Fig. 1A) and CAT (Fig. 1B) in the renal tissues were increased significantly in the As2O3+AST-treated rats as compared with those from the As2O3-exposed rats.
The effect of AST on the renal MDA levels is shown in Fig. 1C. A significant increase in the renal MDA levels was noted after As2O3 treatment. Pretreatment with AST resulted in a significant decrease in the MDA level relative to the As-exposed rats, suggesting a beneficial role of AST on lipid peroxidation.
The ROS levels in the renal tissues were increased significantly in the As2O3 group and As2O3+AST groups when compared with the control group (Fig. 2A). AST treatment significantly decreased the renal ROS levels under basal conditions when compared with the As2O3 groups.
A significant difference was found in 8-OHdG values between As2O3 and normal kidney samples (P < 0.01) (Fig. 2B). However, pretreatment with AST resulted in a significant decrease in the 8-OHdG level relative to that seen in the As2O3-exposed rats, and was dose-dependent.
The levels of total sulfydryl and Na+-K+ ATPase in the As2O3-treated group decreased due to As2O3 exposure (Table 2) compared with the control group (P < 0.01). However, AST treatment attenuated the decrease when compared with the As2O3 group in a dose dependent manner.
The biochemical alterations mentioned above could be correlated with the histological changes in the kidney, as shown in Fig. 4. Histopathological analyses of the kidneys in the control group revealed a normal structure with a regular arrangement of the renal cells (Fig. 4A). In the As2O3-treated rats, the kidneys exhibited severe pathological alterations: renal interstitial hemorrhage, focal necrosis, expansion and hyperemia in renal capsule, plasmolysis of epithelium, and cellular swelling of the renal tubular cell (Fig. 4B). The kidneys of the As2O3-treated rats fed with AST (Fig. 4C-E) showed reduced effects of As2O3 toxicity on renal tissues in a dose dependent manner.
The effects of pretreatment with AST on As concentrations in the kidneys are shown in Fig. 5. Exposure to As2O3 resulted in a significant increase in the As2O3 concentration of the kidneys relative to the control (P < 0.01). AST administration significantly reduced the As accumulation in the kidneys, and the protective effect was dose-dependent.
The serum levels of AST in the high-dose group (200,000 ppm AST-rich biomass of H. pluvialis) during an early period of exposure (at the end of 2 days or 8 days) have been shown to be similar to those noted at the end of 31 days or 91 days [28]. These findings suggest that the duration of exposure to AST is not related to the plasma concentration. AST bioavailability is very low, and it is unlikely to accumulate in the body [29]. Hence, in the present study, the duration of oral administration of AST was 5 days.
Many organisms possess well-developed systems of antioxidant defense to maintain the cellular redox state, including antioxidant enzymes and non-enzymatic antioxidants. Oxidative stress occurs if oxygen free radicals are produced at the levels exceeding those capable of being sequestered by normal cellular antioxidant defenses [30].
For most individuals, the diet is the largest source of exposure to iAsIII [31]. It is well known that iAsIII usually produces excessive ROS, either directly or indirectly, in organ pathophysiology. As a result, the counterbalancing effect of antioxidant enzymes is lost, leading to a depletion of antioxidant enzyme activities. SOD and CAT are major antioxidant enzymes that can protect the body from oxidative damage. GSH is a tripeptide composed of glutamate, cysteine, and glycine. GSH is the most abundant cellular non-protein thiol found at particularly high concentrations in the kidney. GSH acts as an antioxidant, either directly (by scavenging free radicals) or indirectly (by serving as a cofactor for antioxidant enzymes). Because of its abundance, GSH has a crucial role in cellular defense mechanisms against toxicity and oxidative stress, resulting from exposure to toxic compounds and heavy metals [32]. High intracellular levels of GSH confer resistance to As2O3 (at least in part) through the detoxification of ROS [33]. In this study, the GSH/GSSG ratio, as well as levels of GSH, SOD, and CAT, decreased significantly; whereas the levels of ROS and MDA increased in the kidneys of the As2O3-treated rats. These findings suggest that the antioxidant system changed significantly after As2O3 treatment. All of these perturbations in the antioxidant system were attenuated by AST administration. This study showed that the maintenance of antioxidant activity in the body is a possible method for AST preventing oxidative damage and renal injury caused by As2O3.
In general, oxidative stress (including ROS) can damage cellular macromolecules. IAs causes DNA damage by generating ROS and enhancing lipid peroxidation levels [34, 35]. 8-OHdG is one of the major products of ROS-induced DNA damage. It is used widely as an important biomarker of oxidative DNA damage. This study showed that the promotion of repair of oxidative DNA damage can be an important mechanism in the protective effect elicited by AST.
The mitochondrion is the "cellular powerhouse" due to ATP synthesis by oxidative phosphorylation. Mitochondrion is also a major endogenous source of ROS under normal or pathological conditions [23]. Oxidative damage in mitochondria induces a decline in the efficiency of oxidative phosphorylation, and results in an induction of the mitochondrial permeability transition and a release of the pro-apoptotic factors that trigger apoptosis [36]. As2O3 has been shown to induce a decrease in the intracellular levels of GSH, as well as to increase ROS and decrease mitochondrial membrane potential [37]. AST is effective in improving mitochondrial function through retention of the mitochondria in a reduced state under oxidative challenge [38]. Hence, the protective effects of AST on the renal cells may be derived from direct or indirect effects on the mitochondria.
iAsIII is a water-soluble compound that is readily and extensively absorbed from the gastrointestinal tract. Most of them are eliminated from the body by urinary and fecal excretion of non-methylated As and methylated As in the forms of MMA and DMA. However, some accumulate in various organs, such as the kidneys, lungs, liver, heart and spleen. Methylation is important in the distribution in the tissues and excretion of iAs. It has been demonstrated that inhibition of As methylation results in increased tissue concentrations of As [39]. Methyltransferases are needed for methylation, and arsenite methyltransferases from mouse tissues have demonstrated that the testes have the greatest methylation activity, followed by the kidneys, liver, and lungs [40]. In addition, methylation of iAs has demonstrated that S-adenosylmethionine and GSSG are required as methyl donors [41,42]. iAsIII also reacts and binds to the sulfhydryl groups. The total levels of As in the kidney have been considered to be acceptable biological markers of exposure and methylation capacity [43]. The kidney is the main organ for iAs elimination from the body, and accordingly, is a major target for the accumulation and toxicity of iAs [44].
AsIII in As2O3 is a biologically important form of iAs. A novel finding in this study was that AST treatment affected the accumulation of As in the kidney. Co-administration of AST caused a low level of As in the renal tissue, suggesting that AST treatment alleviates iAsIII-induced nephrotoxicity by enhancing the methylation capacity and facilitating iAs excretion. These findings might be related to the restorative effect of AST on the level of GSSG, sulfhydryl groups and S-adenosylmethionine, methyltransferase activity, and methylation efficiency in rats exposed to As2O3.
It has been reported that the four oxygen atoms in each AST ring can coordinate with metal ions, such as CuII and FeIII [45]. Although As is classed as a metalloid, we can not exclude the possibility that the protective effects of AST against As2O3 involve a binding with iAsIII.
ATPase is present in the membranes of the cells and organelles. ATP degradation is an essential process for survival, and ATP is catalyzed by ATPase. As a key enzyme of the transport of biofilm material, energy conversion, and information transfer, ATPase is very sensitive to iAs. iAs can act directly on ATPase, inhibit its activity, and affect the function of transport in the cell membrane. The active site of ATPase contains sulfydryl; iAs bind to it and inactivate the enzyme. A redundant ROS, induced by As2O3, can affect the structure and function of the mitochondria, and result in a reduction of ATPase synthesis. In this study, AST treatment normalized the activities of ATPase in the kidneys of rats exposed to As2O3 in a dose-dependent manner. The greater the consumption of O2, the greater the degradation of the molecule. The reduction in ATPase activity by As2O3 might be linked to a low consumption of O2 uptake in adverse conditions [46]. High levels of As prevent oxygen uptake by the cells, and As accumulation prohibits cellular respiration [47]. AST might protect against the reduction of ATPase activity induced by As2O3 through a modification of cellular respiration in the kidney.
The elevation in the levels of BUN and CREA in the sera of As2O3-treated rats is considered to be an important marker of renal dysfunction [21]. Low clearance values for CREA and BUN indicate a diminished ability of the kidneys to filter these waste products from the blood and excrete them in the urine [20]. Determination of the levels of TP, LDH and ALP, as well as NAG activity in urine, is important for the detection of renal damage in rats. High levels of TP, LDH and ALP, as well as NAG activity, result from increased breakdown of the renal tissues or impaired excretion [21]. The results of these biological indices further suggested that AST treatment significantly ameliorated the renal injury induced by As2O3. In addition, microscopic examinations showed that AST alleviated the histopathological damage in the renal tissues of rats exposed to As2O3.
In conclusion, we demonstrated that the protection against oxidative damage, changes in As accumulation, renal antioxidant defense system, and histopathological damage caused in the kidneys of Wistar rat by As2O3 might be achieved by AST. These findings might be of therapeutic benefit in people or animals suffering from exposure to iAsIII from natural sources or cancer therapy. Because of a few differences between humans and laboratory rats, the obtained results cannot be applied directly to clinical practice. Hence, more intensive studies (in other non-rodent species and humans) are necessary.
Figures and Tables
Fig. 1
Effect of AST and As2O3 on activities of SOD (A), CAT (B), and MDA levels (C) in renal tissues. Values represent the mean ± SE, for 8 rats in each group. *P < 0.01 vs the control group, #P < 0.05 vs the As2O3-treated group (As2O3: 5 mg/kg BW). L: AST: 10 mg/kg BW, As2O3: 5 mg/kg BW. M: AST: 20 mg/kg BW, As2O3: 5 mg/kg BW. H: AST: 40 mg/kg BW, As2O3: 5 mg/kg BW.
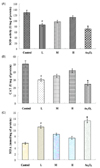
Fig. 2
Effect of AST and As2O3 on ROS (A) and 8-OHdG (B) levels in renal tissues. Values represent the mean ± SE, for 8 rats in each group. *P < 0.01 vs the control group, #P < 0.05 vs the As2O3-treated group (As2O3: 5 mg/kg BW). L: AST: 10 mg/kg BW, As2O3: 5 mg/kg BW. M: AST: 20 mg/kg BW, As2O3: 5 mg/kg BW. H: AST: 40 mg/kg BW, As2O3: 5 mg/kg BW.
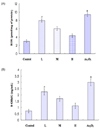
Fig. 3
Effects of AST and As2O3 on the levels of GSH (A) and ratios of GSH/GSSG (B) in renal tissues. Values represent the mean ± SE, for 8 rats in each group. *P < 0.01 vs the control group, #P < 0.05 vs the As2O3-treated group (As2O3: 5 mg/kg BW). L: AST: 10 mg/kg BW, As2O3: 5 mg/kg BW. M: AST: 20 mg/kg BW, As2O3: 5 mg/kg BW. H: AST: 40 mg/kg BW, As2O3: 5 mg/kg BW.
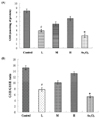
Fig. 4
Hematoxylin and eosin-stained kidney slices from the treated rats. The control rats (A) with normal morphology. The As2O3-treated rats (B) with damaged kidney structure. The As2O3-treated rats fed with AST (C-E) with reduced effects of As2O3 toxicity on the renal tissues. The As2O3+AST-treated rats (AST: 10 mg/kg BW; As2O3: 5 mg/kg BW) (C), As2O3+AST-treated rats (AST: 20 mg/kg BW; As2O3: 5 mg/kg BW) (D), As2O3+AST-treated rats (AST: 40 mg/kg BW; As2O3: 5 mg/kg BW) (E). Original magnification: 200×.
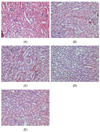
Fig. 5
Effect of AST and As2O3 on the total As accumulation in renal tissues. Values represent the mean ± SE, for 8 rats in each group. *P < 0.01 vs the control group, #P < 0.05 vs the As2O3-treated group (As2O3: 5 mg/kg BW). L: AST: 10 mg/kg BW, As2O3: 5 mg/kg BW. M: AST: 20 mg/kg BW, As2O3: 5 mg/kg BW. H: AST: 40 mg/kg BW, As2O3: 5 mg/kg BW.
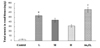
Notes
This work was funded by the National Science Foundation Committee of China (31101868), National Undergraduate Training Programs for Innovation (2012102 24010), the Special Foundation of Postdoctoral Science Foundation of China (2012T50302), and Program for New Century Excellent Talents in Heilongjiang Provincial University (1253-NCET-007).
References
1. Mortensen A, Skibsted LH, Truscott TG. The interaction of dietary carotenoids with radical species. Arch Biochem Biophys. 2001; 385:13–19.


2. Liu X, Osawa T. Astaxanthin protects neuronal cells against oxidative damage and is a potent candidate for brain food. Forum Nutr. 2009; 61:129–135.


3. Lennikov A, Kitaichi N, Fukase R, Murata M, Noda K, Ando R, Ohguchi T, Kawakita T, Ohno S, Ishida S. Amelioration of ultraviolet-induced photokeratitis in mice treated with astaxanthin eye drops. Mol Vis. 2012; 18:455–464.
4. Baig JA, Kazi TG, Shah AQ, Afridi HI, Kandhro GA, Khan S, Kolachi NF, Wadhwa SK, Shah F, Arain MB, Jamali MK. Evaluation of arsenic levels in grain crops samples, irrigated by tube well and canal water. Food Chem Toxicol. 2011; 49:265–270.


5. Horner NS, Beauchemin D. The effect of cooking and washing rice on the bio-accessibility of As, Cu, Fe, V and Zn using an on-line continuous leaching method. Anal Chim Acta. 2013; 758:28–35.


7. Yen YP, Tsai KS, Chen YW, Huang CF, Yang RS, Liu SH. Arsenic induces apoptosis in myoblasts through a reactive oxygen species-induced endoplasmic reticulum stress and mitochondrial dysfunction pathway. Arch Toxicol. 2012; 86:923–933.


8. Henkler F, Brinkmann J, Luch A. The role of oxidative stress in carcinogenesis induced by metals and xenobiotics. Cancer. 2010; 2:376–396.


9. Dwivedi N, Flora SJ. Concomitant exposure to arsenic and organophosphates on tissue oxidative stress in rats. Food Chem Toxicol. 2011; 49:1152–1159.


10. Varol E, Varol S. Effect of fluoride toxicity on cardiovascular systems: role of oxidative stress. Arch Toxicol. 2012; 86:1627.


11. Das TK, Mani V, De S, Banerjee D, Mukherjee A, Polley S, Kewalramani N, Kaur H. Effect of vitamin E supplementation on mRNA expression of superoxide dismutase and interleukin-2 in arsenic exposed goat leukocytes. Bull Environ Contam Toxicol. 2012; 89:1133–1137.


12. Zhang W, Xue J, Ge M, Yu M, Liu L, Zhang Z. Resveratrol attenuates hepatotoxicity of rats exposed to arsenic trioxide. Food Chem Toxicol. 2013; 51:87–92.


13. Naguib YM. Antioxidant activities of astaxanthin and related carotenoids. J Agric Food Chem. 2000; 48:1150–1154.


14. Kurashige M, Okimasu E, Inoue M, Utsumi K. Inhibition of oxidative injury of biological membranes by astaxanthin. Physiol Chem Phys Med NMR. 1990; 22:27–38.
15. Kosanić M, Manojlović N, Janković S, Stanojković T, Ranković B. Evernia prunastri and Pseudoevernia furfuraceae lichens and their major metabolites as antioxidant, antimicrobial and anticancer agents. Food Chem Toxicol. 2013; 53:112–118.


16. Soignet SL, Frankel SR, Douer D, Tallman MS, Kantarjian H, Calleja E, Stone RM, Kalaycio M, Scheinberg DA, Steinherz P, Sievers EL, Coutré S, Dahlberg S, Ellison R, Warrell RP Jr. United States multicenter study of arsenic trioxide in relapsed acute promyelocytic leukemia. J Clin Oncol. 2001; 19:3852–3860.


17. Zhang XW, Yan XJ, Zhou ZR, Yang FF, Wu ZY, Sun HB, Liang WX, Song AX, Lallemand-Breitenbach V, Jeanne M, Zhang QY, Yang HY, Huang QH, Zhou GB, Tong JH, Zhang Y, Wu JH, Hu HY, de The H, Chen SJ, Chen Z. Arsenic trioxide controls the fate of the PML-RARalpha oncoprotein by directly binding PML. Science. 2010; 328:240–243.


18. Chen SJ, Zhou GB, Zhang XW, Mao JH, de Thé H, Chen Z. From an old remedy to a magic bullet: molecular mechanisms underlying the therapeutic effects of arsenic in fighting leukemia. Blood. 2011; 117:6425–6437.


19. Nandi D, Patra RC, Swarup D. Effect of cysteine, methionine, ascorbic acid and thiamine on arsenic-induced oxidative stress and biochemical alterations in rats. Toxicology. 2005; 211:26–35.


20. Yousef MI, El-Demerdash FM, Radwan FM. Sodium arsenite induced biochemical perturbations in rats: ameliorating effect of curcumin. Food Chem Toxicol. 2008; 46:3506–3511.


21. Augusti PR, Conterato GM, Somacal S, Sobieski R, Spohr PR, Torres JV, Charão MF, Moro AM, Rocha MP, Garcia SC, Emanuelli T. Effect of astaxanthin on kidney function impairment and oxidative stress induced by mercuric chloride in rats. Food Chem Toxicol. 2008; 46:212–219.


22. Curek GD, Cort A, Yucel G, Demir N, Ozturk S, Elpek GO, Savas B, Aslan M. Effect of astaxanthin on hepatocellular injury following ischemia/reperfusion. Toxicology. 2010; 267:147–153.


23. Lee DH, Kim CS, Lee YJ. Astaxanthin protects against MPTP/MPP+-induced mitochondrial dysfunction and ROS production in vivo and in vitro. Food Chem Toxicol. 2011; 49:271–280.


24. Cui X, Li S, Shraim A, Kobayashi Y, Hayakawa T, Kanno S, Yamamoto M, Hirano S. Subchronic exposure to arsenic through drinking water alters expression of cancer-related genes in rat liver. Toxicol Pathol. 2004; 32:64–72.


25. Lakritz J, Plopper CG, Buckpitt AR. Validated high-performance liquid chromatography-electrochemical method for determination of glutathione and glutathione disulfide in small tissue samples. Anal Biochem. 1997; 247:63–68.


26. Socci DJ, Bjugstad KB, Jones HC, Pattisapu JV, Arendash GW. Evidence that oxidative stress is associated with the pathophysiology of inherited hydrocephalus in the H-Tx rat model. Exp Neurol. 1999; 155:109–117.


27. Jain A, Yadav A, Bozhkov AI, Padalko VI, Flora SJ. Therapeutic efficacy of silymarin and naringenin in reducing arsenic-induced hepatic damage in young rats. Ecotoxicol Environ Saf. 2011; 74:607–614.


28. Stewart JS, Lignell A, Pettersson A, Elfving E, Soni MG. Safety assessment of astaxanthin-rich microalgae biomass: acute and subchronic toxicity studies in rats. Food Chem Toxicol. 2008; 46:3030–3036.


29. Petri D, Lundebye AK. Tissue distribution of astaxanthin in rats following exposure to graded levels in the feed. Comp Biochem Physiol C Toxicol Pharmacol. 2007; 145:202–209.


30. Trpkovic A, Todorovic-Markovic B, Trajkovic V. Toxicity of pristine versus functionalized fullerenes: mechanisms of cell damage and the role of oxidative stress. Arch Toxicol. 2012; 86:1809–1827.


31. Oguri T, Yoshinaga J, Tao H, Nakazato T. Daily intake of inorganic arsenic and some organic arsenic species of Japanese subjects. Food Chem Toxicol. 2012; 50:2663–2667.


32. Chen Y, Krishan M, Nebert DW, Shertzer HG. Glutathione-deficient mice are susceptible to TCDD-induced hepatocellular toxicity but resistant to steatosis. Chem Res Toxicol. 2012; 25:94–100.


33. Bhalla S, Gordon LI, David K, Prachand S, Singh AT, Yang S, Winter JN, Guo D, O'Halloran T, Platanias LC, Evens AM. Glutathione depletion enhances arsenic trioxide-induced apoptosis in lymphoma cells through mitochondrial-independent mechanisms. Br J Haematol. 2010; 150:365–369.


34. Qin XJ, Hudson LG, Liu W, Ding W, Cooper KL, Liu KJ. Dual actions involved in arsenite-induced oxidative DNA damage. Chem Res Toxicol. 2008; 21:1806–1813.


35. Zhou X, Sun X, Cooper KL, Wang F, Liu KJ, Hudson LG. Arsenite interacts selectively with zinc finger proteins containing C3H1 or C4 motifs. J Biol Chem. 2011; 286:22855–22863.


36. Seo AY, Joseph AM, Dutta D, Hwang JC, Aris JP, Leeuwenburgh C. New insights into the role of mitochondria in aging: mitochondrial dynamics and more. J Cell Sci. 2010; 123:2533–2542.


37. Cheng B, Yang X, An L, Gao B, Liu X. Arsenic trioxide-induced apoptosis of Hep-2 cell line through modulating intracellular glutathione (GSH) level. Auris Nasus Larynx. 2010; 37:89–94.


38. Wolf AM, Asoh S, Hiranuma H, Ohsawa I, Iio K, Satou A, Ishikura M, Ohta S. Astaxanthin protects mitochondrial redox state and functional integrity against oxidative stress. J Nutr Biochem. 2010; 21:381–389.


39. Marafante E, Vahter M, Envall J. The role of the methylation in the detoxication of arsenate in the rabbit. Chem Biol Interact. 1985; 56:225–238.


40. Healy SM, Casarez EA, Ayala-Fierro F, Aposhian H. Enzymatic methylation of arsenic compounds. V. Arsenite methyltransferase activity in tissues of mice. Toxicol Appl Pharmacol. 1998; 148:65–70.
41. Lin S, Shi Q, Nix FB, Styblo M, Beck MA, Herbin-Davis KM, Hall LL, Simeonsson JB, Thomas DJ. A novel S-adenosyl-L-methionine: arsenic(III) methyltransferase from rat liver cytosol. J Biol Chem. 2002; 277:10795–10803.


42. Leslie EM. Arsenic-glutathione conjugate transport by the human multidrug resistance proteins (MRPs/ABCCs). J Inorg Biochem. 2012; 108:141–149.


43. Chiou HY, Hsueh YM, Hsieh LL, Hsu LI, Hsu YH, Hsieh FI, Wei ML, Chen HC, Yang HT, Leu LC, Chu TH, Chen-Wu C, Yang MH, Chen CJ. Arsenic methylation capacity, body retention, and null genotypes of glutathione S-transferase M1 and T1 among current arsenic-exposed residents in Taiwan. Mutat Res. 1997; 386:197–207.


45. Zhao L, Chen F, Zhao G, Wang Z, Liao X, Hu X. Isomerization of trans-astaxanthin induced by copper(II) ion in ethanol. J Agric Food Chem. 2005; 53:9620–9623.


46. Haque MS, Roy SK, Shahjahan M. Arsenic impairs the effect of low temperature on the regulation of Na+-K+ ATPase activity in skeletal muscle of fish (Channa punctata). Turkish J Fish Aquat Sci. 2011; 11:339–344.