Abstract
Oral administration of royal jelly (RJ) promotes wound healing in diabetic mice. Concerns have arisen regarding the efficacy of RJ on the wound healing process of normal skin cells. In this study, a wound was created by scratching normal human dermal fibroblasts, one of the major cells involved in the wound healing process. The area was promptly treated with RJ at varying concentrations of 0.1, 1.0, or 5 mg/ml for up to 48 hrs and migration was analyzed by evaluating closure of the wound margins. Furthermore, altered levels of lipids, which were recently reported to participate in the wound healing process, were analyzed by HPTLC and HPLC. Migration of fibroblasts peaked at 24 hrs after wounding. RJ treatment significantly accelerated the migration of fibroblasts in a dose-dependent manner at 8 hrs. Although RJ also accelerated the migration of fibroblasts at both 20 hrs and 24 hrs after wounding, the efficacy was less potent than at 8 hrs. Among various lipid classes within fibroblasts, the level of cholesterol was significantly decreased at 8 hrs following administration of both 0.1 ug/ml and 5 mg/ml RJ. Despite a dose-dependent increase in sphinganines, the levels of sphingosines, ceramides, and glucosylceramides were not altered with any concentration of RJ. We demonstrated that RJ enhances the migration of fibroblasts and alters the levels of various lipids involved in the wound healing process.
Royal jelly (RJ), a secretion of the hypo-pharyngeal and mandibular glands of worker honey bees, is a health tonic widely consumed with various perceived benefits [1]. RJ has been used since ancient times as a remedy for wound healing [2]. However, although the results from a randomized clinical trial document that RJ accelerates wound healing, no study has been performed regarding the influence of RJ on human skin cells, including dermal fibroblasts. Several studies demonstrate that oral administration of RJ promotes wound healing in diabetic mice through anti-inflammatory effects and by enhancing the formation of granular tissue. Concerns have arisen regarding the efficacy of RJ in the wound healing process of normal skin cells. Through intensive studies on various in vitro and animal models, the wound healing enhancing effects of RJ have been demonstrated [2-8]. Wound healing of the skin is a complex process that involves an inflammatory response, new tissue formation, and remodeling of the extracellular matrix [3]. After the initial inflammatory response, new tissue formation begins with migration and proliferation of keratinocytes at the wound edge, followed by migration of dermal fibroblasts into the area of the wound where they proliferate and deposit extracellular matrix [9]. Throughout the wound healing process, fibroblasts, as well as inflammatory cells, produce various growth factors, cytokines, and mitogens that play intertwined and important roles in wound closure [9]. Recent evidence indicates that increased lipid biosynthesis may also enhance wound healing closure and restoration of the barrier function of the skin during the wound healing process. The skin barrier is formed from the lipid-enriched membrane of the stratum corneum, which is the outer most layer of the epidermis, and is organized with a repetitive lamellar structure of sphingolipids, cholesterol, and fatty acids [3,10]. Reduced lipid synthesis delays wound healing [11] and enhanced wound closure with an increased level of skin barrier lipids has been reported during the wound healing process of skin [11,12].
In this study, we investigate if RJ treatment accelerates the migration of dermal fibroblasts, one of the major cells involved in granular tissue formation during wound healing. Furthermore, we determine whether RJ treatment alters the levels of lipids in fibroblast; such alterations have recently been reported to aid in wound closure. Our data presented herein demonstrate that RJ enhances the migration of fibroblasts and alters the levels of various lipids involved in the wound healing process.
Normal human dermal fibroblast (neonatal; C-004-5C) (HDFn) growth supplement was purchased from Cascade Biologics (Portland, OR, USA). Cell culture media including Dulbecco's modified Eagle's medium (DMEM) and fetal bovine serum (FBS) were obtained from Gibco BRL (Gathersburg, MD, USA). Mytocin C was purchased from Sigma-Aldrich (St. Louis, MO, USA). High-performance thin layer chromatography (HPTLC) plates (Silca Gel 60) were purchased from Merck (Dermstadt, Germany). High-performance liquid chromatography (HPLC; Agilent 1100 series) was performed using a C18 reversed-phase column (symmetry C18 5 µm 4.6 mm × 150 mm column; Milford MA. USA).
RJ collected in Korea was used throughout the experiments. The RJ was suspended in sterile phosphate-buffered saline (PBS). Prior to treatment, the cells were dissolved in a DMSO concentration at 1 mg/ml. The RJ suspension was then divided into aliquots and stored at -80℃ until administration. No bacteria colony formation was observed in these RJ suspensions as examined by a standard agar plate culture assay [13]. The endotoxin content of the RJ was < 50 pg/mg as measured by a Limulus amebocyte lysate assay (Seikagaku Kogyo, Tokyo, Japan) [14].
Fibroblasts: Human dermal fibroblasts (HDFn) were isolated from neonatal foreskin. Primary cultures of HDFn were grown from human dermal fibroblast tissue removed at the time of reduction mammoplasty and were used at passage 2-15 in primary cultures of diabetic mice. Fibroblasts were prepared from the diabetic mice and were used at passage 3-10. The HDFn were maintained in DMEM containing 10% fetal bovine serum (FBS) (Gibco, Grand Island, NY) and 1% penicillin-streptomycin. Cells were incubated at 37℃ in 5% CO2 [15,16].
Cytotoxicity was assessed using a 3-[4,5-dimethylthiazol-2-yl]-2,5-diphenyltetrazolium bromide (MTT) assay [17,18]. HDFn cells were seeded in 96-well plates and treated with various concentrations of RJ. They were cultured in a 5% CO2 incubator for the indicated periods. After removing the media, MTT (0.5 mg/ml) was added and incubated at 37℃ for 2 hrs. Supernatants were carefully discarded and 1 ml of DMSO was added. After incubating at 25℃ for 30 min, the reactants were measured by a microplate reader (UV max, Molecular Devices, USA) at 570 nm.
Cells were grown to 60% confluence in 6-well plates. To eliminate cell proliferation, all cells were pretreated with 10 ug/ml mitomycin C (Sigma, MO) for 1 hr and washed with PBS prior to scratching. The scratchings were performed by scraping with a sterile rubber policeman. The wound margins were photographed at time t = 0 under a microscope (Motic AE31: Motic Instrument Inc, Richmond, BC, Canada) using a digital camera (Moticam 1300: Motic Instrument Inc, Richmond, BC, Canada) [19]. Cells were treated for up to 48 hrs with cell media only (control) or with 0.1 ug/ml, 1 ug/ml, or 5 ug/ml of RJ dissolved in cell media. The wounds were subsequently re-photographed in the same field at different time points. The experiments were performed in triplicate for each of four independent trials. Cell migration was analyzed by image J (image processing and analysis in Java by NIH image: http://rsb.info.nih.govij/) and expressed as percent wound coverage by cells moving into the scratched wound area at different time points. The concentration range of RJ (0.1-5 ug/ml) was based on RJ concentration with > 90% cell viability by MTT assay (Promega, Madison, WI, USA) in a preliminary experiment (data not shown). Cell migration data were expressed as percent wound coverage.
The cells in the wounds were treated for 8 hrs with RJ at varying concentrations of 0.1 ug/ml, 1 ug/ml, or 5 ug/ml. The treated cells were placed in 0.24% HCl in methanol and crushed with a polytron. The resulting solution (chloroform/methanol, 1:1 v/v) was combined with the 3 N NaOH supernatant and the sphingosine content was analyzed. The component lipids were then separated using HPTLC on 10 × 10 cm silica gel plates (Merck, Darmastdt, Germany). To aid in separation, the lower portion was added to a mixture of choloroform: 1 M NaCl (1:1, v/v) and 3 N NaOH. The lower part of the solution containing 0.15 M KOH in methanol was then added. HPTLC analysis of the lower portion of the generated mixture was used in this experiment.
Ceramide, glucosylceramide (GC), triglyceride (TG), and cholesterol deployment conditions were as follows: the spotting volume was 20 ul for all lanes; the plates were chromatograph in the 10 cm dimension; the receptacles were TLC tanks (6 × 12 × 3.75 cm in; Analabs, North Haven, CT) lined with filter paper; solvent systems (approximately 100 ml total) were equilibrated several hours before use; and lipids were measured by spotting lipid extracts and lipid standards (Sigma. Co., St. Louis. MO.) on the plates and separating them using a semi-automatic operator (Limonat 5, Merck, Darmaster, Germany). The first development was carried out in a chloroform: methanol: H2O (40:10:1, v/v/v) mixture until the solvent front had migrated approximately 2.5 cm. Following development, excess solvent was evaporated in a fume hood for 15 min and then in a vacuum desiccator for 15 min. The second development was performed in a chloroform: methanol: acetic acid (40:10:1, v/v/v) mixture until the solvent front had migrated approximately 5 cm. The second development was repeated (third development). The front development was carried out in n-hexane: diethyl ether: acetic acid (65:35:1, v/v/v) until the solvent front had migrated approximately 8.5 cm [20]. Excess solvent was evaporated as described above. Plates were sprayed with a 0.25% sodium dichromate (w/v) 15% sulfuric acid (v/v) reagent and heated for 30 min at 120℃ and then dipped into a 3% cupric acetate (w/v) 8% phosphoric acid (v/v) solution and either heated at 180℃ for 15 min or at 130℃ for 30 min. All plates were either heated on an aluminum block (20 × 20 × 2.5 cm) set on a hot plate (130 ℃) or placed in a hot air oven (180℃). Plates were either scanned immediately after heating, or stored at -20℃ and scanned within 24 hrs. Finally, the HPTLC plates were cooled to room temperature and the lipid samples were quantified by photodensitometry at 254 nm using the CAMAG HPTLC scanner III (CAMAG, Muttenz, Switzerland). Analytic data were processed by computer using the CAMAG HPTLC program (WINCAT software, version 2.2) [21].
The hydroxyl fatty acids from the HDFn homogenate were placed in an ice-cold chloroform:methanol (2:1, v/v) solution after acidification to a pH of 3.0. Next, 10 ml of a RIPA buffer (20 mM Tris-HCl, pH 3.0, 150 mM NaCl, 10 mM NaPO4, 10% glycerol, 10M Na3VO4, 100M ammonium molybdate, 1% NP-40, 0.1% SDS) was added to the HDFn. This mixture was then pulverized with a polytron. An equivalent amount of Folch solution [22] was added to the pulverized samples, the resulting mixture was then centrifuged (3,000 rpm, 5 min). The lower part of the solution was collected, dried with nitrogen gas, and dissolved in 20 ul of methanol. The hydroxyl fatty acids from the HDFn were then subjected to reverse phase HPLC (RP-HPLC) using a Beckman 5 um octadecylsilica (ODS 18) column (symmetry C18 5 µm 4.6 mm × 150 mm column; Milford MA. USA) as reported previously [23]. The chromatographic system was run isocratically at a flow rate of 1.0 ml/min on a Waters Breeze HPLC system (515 HPLC pump with UV/vis Detector, Waters, Inc., U.S.A.). The mobile phase solvents were 74% methanol and 26% H2O containing 0.8 g/L acetic acid. A photo-diode array detector with a UV absorption spectrum (2996 PDA Dectector, Waters, Co, U.S.A.) was used to confirm the purification of separated hydroxyl fatty acids from each sample in the presence of an analytically useful absorption band, with Amax = 238 nm (€ 238 = 28,000/M/cm). The level of hydroxyl fatty acids in each sample was quantified using external standards of sphinganine and sphingosine. A calibration curve with the various concentrations of the external standards revealed good linearity, with R2 values exceeding 0.99 (peak area vs concentration). The detection limit for each external standard was 2 mg/L.
When the in vitro migration assay was performed with fibroblasts, > 100% of wound coverage was achieved with fibroblasts (Fig. 2) at 48 hrs after wound scratching. When 1 ug/ml or 5 ug/ml RJ was applied for 8 hrs after wound scratching, wound coverage was significantly increased for HDFn (data not shown; 79.4 ± 3.6 for 1 ug/ml RJ; 78.4 ± 5.4 for 5 ug/ml RJ) as compared to the medium-only treated control group. However, after 4 hrs wound coverage for HDFn treated with 0.1 ug/ml, 1 ug/ml, or 5 ug/ml RJ was similar to that of the control group. Additionally, overall wound coverage in HDFn (70-80%) after 48 hrs of treatment with 0.1 ug/ml, 1 ug/ml, or 5 ug/ml RJ was similar to that of the control group.
We showed that lipid composition in HDFn was altered by RJ. When the lipid classes within the fibroblasts were assessed at 8 hrs after treatment with RJ, the level of cholesterol revealed the most noteworthy change in wound coverage in the groups treated with 0.1 ug/ml and 5 ug/ml RJ (Fig. 3A). Although TG tended to decrease with 0.1 ug/ml and 5 ug/ml RJ, no significant difference was observed. Furthermore, no significant changes were noted compared to the control with regard to the levels of ceramides, glycosylceramides, and TG following treatment with 0.1 ug/ml, 1 ug/ml, or 5 ug/ml RJ (Fig. 3A). We next used RP-HPLC to analyze HDFn following 8 hrs of treatment with RJ. No changes in the sphingosine level were noted with any concentration of RJ at 8 hrs (Fig. 3B). However, we did observe a dose-dependent increase in the level of sphinganine. This increase in sphinganine appeared to have the greatest effect on wound coverage following application of RJ at 5 ug/ml. In our experiment, the observed increase in sphinganine (sphingoid bases: sphingosine and sphinganine) was likely due to an up regulation in sphingolipid metabolism, in particular with the enzyme that catalyzes the condensation of sphinganine and fatty acyl-CoA to form dihydroceramides, which are rapidly oxidized to form lipids. This increase in the level of sphinganines appears to play a role in the wound-coverage enhancing effects of RJ in HDFn. This result, in conjunction with the observed decrease in cholesterol content, demonstrates a dose-dependent effect of RJ in the wound healing process (Fig. 2 and 3).
In this study, the cutaneous effects of RJ were evaluated in an in vitro wound produced by a sterile scraper. We investigated the effect of RJ on the migration of human dermal fibroblasts (HDFn). In preliminary dose-dependence experiments using RJ, varying concentrations of 0.1 ug/ml, 1 ug/ml, or 5 ug/ml were shown to have > 100% cell viability by MTT assay (Fig. 1). The migration of fibroblasts is the initial response in the formation of new tissue in the wound healing process of skin [24,25]. Migration was monitored for up to 48 hrs. RJ at concentrations of 0.1 ug/ml, 1 ug/ml, and 5 ug/ml demonstrated improved wound coverage with HDFn at 20 hrs in an in vitro migration assay. Concentrations of RJ at 0.1 ug/ml, 1 ug/ml, and 5 ug/ml were used in the present study given the evidence that they improved wound coverage with HDFn in an in vitro migration assay after 48 hrs (Fig. 2, 3). When 5 ug/ml of RJ was applied for 8 hrs, wound coverage of HDFn was significantly increased compared to the control group (Fig. 2). However, the beneficial effects of RJ were not observed at concentrations of 0.1 ug/ml and 1 ug/ml after 8 hrs. Additionally, these concentrations were not found to significantly alter lipid levels in HDFn after 8 hrs. In contrast, TG and cholesterol levels were significantly decreased in HDFn after treatment with 5 ug/ml of RJ, as compared to the control group (Fig. 3A). Despite a dose-dependent increase in sphinganine, no significant changes in the level of sphingosine were noted with any concentration of RJ after 8 hrs (Fig. 3B). Given the apparent functional importance of alterations in lipid content in facilitating the migration of HDFn during wound healing, we evaluated the lipid composition of HDFn after 8 hrs of treatment with RJ at a concentration of 5 ug/ml. Although the levels of ceramides, glucosylceramides, and TG were not altered, the cholesterol level was observed to be significantly decreased after treatment with 5 ug/ml RJ (Fig. 3A). In contrast to our results, previous reports found that in cultured skin substitutes grown in vitro the levels of cholesterol and free fatty acids were increased during the healing process with grafting [10,26]. An increase in the levels of lipids in HDFn, in particular sphingolipids such as ceramides and glucosylceramides, has been noted to occur in the wound healing process [27,28]. Also, increases in the levels of glucosylceramides and ceramides were highly distinct [26,29]. However, despite a dose-dependent increase in sphinganine, no alterations in sphingosine levels were noted with any concentration of RJ in our investigation (Fig. 3B). This positive association of increased composition of sphingoid bases with enhanced migration of HDFn following treatment with 5 ug/ml RJ is concordant with the results of previous studies of cultured skin substitutes, in which the levels of glucosphingolipids and acylglucosylceramides were increased after the use of grafting in wound healing [30,31]. These findings, together with reports that reductions in lipid composition play a role in wound healing, suggest that an increase in the composition of sphingolipids enhances wound coverage.
Recently, evidence suggests that sphinganine (without a 4,5-trans double bond) has a greater apoptotic potency than sphingosine (with a 4,5-trans double bond). Sphinganine might increase cellular cAMP to induce the differentiation of stem cells into normal adult cells. Additionally, sphingoid bases function as second messengers in signal transduction pathways, inhibiting cell proliferation and inducing apoptosis in human cancer cells [32,33]. It is uncertain whether a sphingosine or sphinganine backbone plays a role in mediating the anti-proliferative and pro-apoptotic activities of ceramide in cancer cells [34,35]. Furthermore, recent evidence suggests that sphingolipids act as intracellular mediators in the wound closure process and help to restore the barrier function of skin. In fact, upon wound formation the intracellular level of a major sphingolipid was increased by the activation of ceramide-generating enzymes, such as sphingomyelinase, in a stress-associated signaling pathway, which in turn increased the expression of peroxisome proliferator-activated receptor β (PPAR β) [36,37]. Concomitantly, wound formation also increases the generation of PPAR β ligands, which include several sphingolipids [31,33,36,38,39]. The resulting increase in the level of PPAR β transcription activity ultimately accelerates the viability, adhesion, and migration of HDFn during the wound healing process [19,26,36,37,40].
Royal jelly (RJ), Apis mellifera Linne, is well known as a valuable food because of its positive nutritional and physiological properties [1,4,6,41]. The principal constituents of RJ include water, protein, sugars, lipids, and mineral salts. Royal jelly is included in many dermatological preparations, but most prominently in those used for skin refreshing and skin regeneration or rejuvenation. It is also used in creams or ointments for healing burns and other wounds. It is usually included in very small dosages (0.05-1%); it is likely that it deteriorates relatively quickly. Several of the substances within RJ are pharmacologically active, including 10-hydroxy-2-decanoic acid (10HDA) [42,43], royalisin [44], and apisin [45]. RJ contains various fatty acids that are similar to 10HDA in molecular structure [42,43,46]. A hydroxyl fatty acid, 10HDA is the primary bioactive component [42,43,47,48] contained in RJ and has been shown to enhance wound healing in multiple intensive studies [2]. This fatty acid induces the fibroblast cell line, HDFn, to produce transforming growth factor-β1 (TGF-β1), which is an important factor for collagen production. The collagen production-promoting activity of 10 HDA can be neutralized by anti-TGF-β1 antibody. As such, several mechanisms have been proposed regarding the anti-inflammatory and anti-proliferative properties of 10HDA. These include increased expression of vascular endothelial growth factor (VEGF) [43], transforming growth factor β-1 (TGF β-1), and fibroblast growth factor (FGF-1), blocking of the mRNA splicing of tumor necrosis factor (TNF-α) and collagenase (MMP-1), and inhibition of the biosynthesis of lipoxygenase and cycloxygenase metabolites [42,43]. TGF β has been shown to act on human dermal fibroblasts at the pretranslational level by stimulating the accumulation of fibronectin and type 1 procollagen mRNA [49]. The sum of the activities exerted by these hydroxyl fatty acids may enhance collagen production and promote wound healing. It has been shown that 10HDA increases collagen production in vivo and in vitro and that it can provide protective anti-oxidative effects in photodamaged skin, increase the level of hyaluronic acid, and increase the quantity and quality of elastin fiber tissue. Propyl hydroxylase and lysyl hydroxylase are the key enzymes in collagen synthesis, and they work by the hydroxylating proline and lysine residues in the procollagen peptide. Although it is well established that 10HDA performs various functions in the skin, if and how influences of other molecules are still in doubt. Proteins and sugars make up the largest fractions of RJ. Proteins account for 73.9% of the nitrogenous substances in RJ and four of the six major proteins [50] are glycoproteins [45]. In a previous study, the glycoprotein fraction was shown to enhance wound healing in hairless mice within 8 days of injury by increasing cell proliferation [51]. RJ also contains monosaccahrides, such as pentose and galactose [50]. Protein-nitrogen makes up a larger percentage of the water-soluble nitrogen in RJ than amino nitrogen. Furthermore, some of the proteins in RJ are glycoproteins that contain mannose and glucose [52]. Carboxylic acids are the primary ether extract components in RJ. As such, RJ may stimulate increased collagen production and enhance the deposition of collagen in the dermis, thus facilitating wound healing.
This study demonstrated the novel finding that a 5 ug/ml concentration of decocted RJ enhances the migration of human fibroblasts and increase the level of sphingolipids in an in vitro wound healing model. To more fully evaluate the role of RJ in wound healing, future investigations will need to explore the effects of various concentrations of RJ on the enhancement of wound healing and sphingolipids content.
Figures and Tables
Fig. 2
RJ induced migration of human dermal fibroblasts in an in vitro wound models. (A) Human dermal fibroblasts were grown to confluence and an in vitro wound was produced using a sterile scraper. 0.1 µg/ml, 1 µg/ml or 5 µg/ml of RJ was treated immediately after wounding. Migration was monitored for up to 48 hrs. Some fields were photographed immediately after wounding. Bold lines represented in vitro scratch and thin lines represent the migration front. A representative experiment is shown. (B) Histograms indicate the average coverage of scratch wounds widths in % relative to baseline wound width at 4, 8 and 20 hrs after Royal Jelly treatments. The % of wound coverage was measured using scan image. Values are mean ± SE (n = 9). Means with different letters at each time differ P < 0.05.
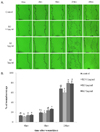
Fig. 3
RJ regulated lipid composition in normal human dermal fibroblasts. Normal human fibroblasts were grown to confluence and an in vitro wound was produced using a sterile scraper. 0.1 µg/ml, 1 µg/ml or 5 µg/ml of RJ was treated for 24 hrs immediately after wounding. Total lipids were extracted and the altered level of lipid composition were analyses by HPTLC (A) and HPLC (B). Values are mean ± SE (n = 9). Means with different letters at each differ P < 0.05. GC ; Glucosylceramide, TG ; Triglyceride.
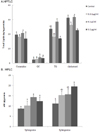
References
1. Viuda-Martos M, Ruiz-Navajas Y, Fernandez-Lopez J, Perez-Alvarez JA. Functional properties of honey, propolis, and royal jelly. J Food Sci. 2008. 73:R117–R124.


2. Fujii A, Kobayashi S, Kuboyama N, Furukawa Y, Kaneko Y, Ishihama S, Yamamoto H, Tamura T. Augmentation of wound healing by royal jelly (RJ) in streptozotocin-diabetic rats. Jpn J Pharmacol. 1990. 53:331–337.


3. Werner S, Grose R. Regulation of wound healing by growth factors and cytokines. Physiol Rev. 2003. 83:835–870.


4. Guo H, Saiga A, Sato M, Miyazawa I, Shibata M, Takahata Y, Morimatsu F. Royal jelly supplementation improves lipoprotein metabolism in humans. J Nutr Sci Vitaminol (Tokyo). 2007. 53:345–348.


6. Salazar-Olivo LA, Paz-Gonzalez V. Screening of biological activities present in honeybee (Apis mellifera) royal jelly. Toxicol In Vitro. 2005. 19:645–651.


7. Munstedt K, Bargello M, Hauenschild A. Royal jelly reduces the serum glucose levels in healthy subjects. J Med Food. 2009. 12:1170–1172.


8. Gasic S, Vucevic D, Vasilijic S, Antunovic M, Chinou I, Colic M. Evaluation of the immunomodulatory activities of royal jelly components in vitro. Immunopharmacol Immunotoxicol. 2007. 29:521–536.


10. Vicanova J, Ponec M, Weerheim A, Swope V, Westbrook M, Harriger D, Boyce S. Epidermal lipid metabolism of cultured skin substitutes during healing of full-thickness wounds in athymic mice. Wound Repair Regen. 1997. 5:329–338.


11. Altavilla D, Saitta A, Cucinotta D, Galeano M, Deodato B, Colonna M, Torre V, Russo G, Sardella A, Urna G, Campo GM, Cavallari V, Squadrito G, Squadrito F. Inhibition of lipid peroxidation restores impaired vascular endothelial growth factor expression and stimulates wound healing and angiogenesis in the genetically diabetic mouse. Diabetes. 2001. 50:667–674.


12. Proksch E. Regulation of the epidermal permeability barrier by lipids and hyperproliferation. Hautarzt. 1992. 43:331–338.
13. Taniguchi Y, Kohno K, Inoue S, Koya-Miyata S, Okamoto I, Arai N, Iwaki K, Ikeda M, Kurimoto M. Oral administration of royal jelly inhibits the development of atopic dermatitis-like skin lesions in NC/Nga mice. Int Immunopharmacol. 2003. 3:1313–1324.


14. Bincoletto C, Eberlin S, Figueiredo CAV, Luengo MB, Queiroz MLS. Effects produced by royal jelly on haematopoiesis: Relation with host resistance against ehrlich ascites tumour challenge. Int Immunopharmacol. 2005. 5:679–688.


15. Witte MB, Barbul A. General proniples of wound healing. Surg Clin North Am. 1997. 77:509–528.
16. Berg EL, Yang J, Melrose J, Nguyen D, Privat S, Rosler E, Kunkel EJ, Ekins S. Chemical target and pathway toxicity mechanisms defined in primary human cell systems. J Pharmacol Toxicol Methods. 2010. 61:3–15.


17. Denizot F, Lang R. Rapid colorimetric assay for cell growth and survival: Modifications to the tetrazolium dye procedure giving improved sensitivity and reliability. J Immunol Methods. 1986. 89:271–277.
18. Mosmann T. Rapid colorimetric assay for cellular growth and survival: Application to proliferation and cytotoxicity assays. J Immunol Methods. 1983. 65:55–63.


19. Lo CM, Buxton DB, Chua GC, Dembo M, Adelstein RS, Wang YL. Nonmuscle myosin IIb is involved in the guidance of fibroblast migration. Mol Biol Cell. 2004. 15:982–989.


20. Bligh EG, Dyer WJ. A rapid method of total lipid extraction and purification. Can J Biochem Physiol. 1959. 37:911–917.


21. Melnik BC, Hollmann J, Erler E, Verhoeven B, Plewig G. Microanalytical screening of all major stratum corneum lipids by sequential high-performance thin-layer chromatography. J Invest Dermatol. 1989. 92:231–234.


22. Folch J, Lees M, Sloane Stanley GH. A simple method for the isolation and purification of total lipids from animal tissues. J Biol Chem. 1957. 226:497–509.


23. Patella V, Bouvet JP, Marone G. Protein fv produced during vital hepatitis is a novel activator of human basophiles and mast cells. J Immunol. 1993. 151:5685–5698.
26. Matuszewska B, Keogan M, Fisher DM, Soper KA, Hoe CM, Huber AC, Bondi JV. Acidic fibroblast growth factor: Evaluation of topical formulations in a diabetic mouse wound healing model. Pharm Res. 1994. 11:65–71.
27. Turkmen Z, Cavusoglu K, Cavusoglu K, Yapar K, Yalcin E. Protective role of royal jelly (honeybee) on genotoxicity and lipid peroxidation, induced by petroleum wastewater, in Allium cepa L. root tips. Environ Technol. 2009. 30:1205–1214.
28. Holleran WM, Feingold KR, Man MQ, Gao WN, Lee JM, Elias PM. Regulation of epidermal sphingolipid synthesis by permeability barrier function. J Lipid Res. 1991. 32:1151–1158.


29. Rother J, Echten Gv, Schwarzmann G, Sandhoff K. Biosynthesis of sphingolipids: Dihydroceramide and not sphinganine is desaturated by cultured cells. Biochem Biophys Res Commun. 1992. 189:14–20.


30. Hakomori S. Bifunctional role of glycosphingolipids. modulators for transmembrane signaling and mediators for cellular interactions. J Biol Chem. 1990. 265:18713–18716.


31. Hannun YA, Bell RM. Functions of sphingolipids and sphingolipid breakdown products in cellular regulation. Science. 1989. 243:500–507.


32. Spiegel S, Merrill AH Jr. Sphingolipid metabolism and cell growth regulation. FASEB J. 1996. 10:1388–1397.


33. Huwiler A, Kolter T, Pfeilschifter J, Sandhoff K. Physiology and pathophysiology of sphingolipid metabolism and signaling. Biochim Biophys Acta. 2000. 1485:63–99.


34. Radin NS. Apoptotic death by ceramide: Will the real killer please stand up? Med Hypotheses. 2001. 57:96–100.


35. Goswami R, Dawson G. Does ceramide play a role in neural cell apoptosis? J Neurosci Res. 2000. 60:141–149.


36. Tan NS, Michalik L, Desvergne B, Wahli W. Peroxisome proliferator-activated receptor (PPAR)-beta as a target for wound healing drugs: What is possible? Am J Clin Dermatol. 2003. 4:523–530.


37. Candela M, Barker SC, Ballou LR. Sphingosine synergistically stimulates tumor necrosis factor alpha-induced prostaglandin E2 production in human fibroblasts. J Exp Med. 1991. 174:1363–1369.


38. Liao J, Tao J, Lin G, Liu D. Chemistry and biology of sphingolipids. Tetrahedron. 2005. 61:4715–4733.


39. Hannun YA, Luberto C, Argraves KM. Enzymes of sphingolipid metabolism: From modular to integrative signaling. Biochemistry. 2001. 40:4893–4903.


40. Brown LF, Lanir N, McDonagh J, Tognazzi K, Dvorak AM, Dvorak HF. Fibroblast migration in fibrin gel matrices. Am J Pathol. 1993. 142:273–283.
41. Takahama H, Shimazu T. Food-induced anaphylaxis caused by ingestion of royal jelly. J Dermatol. 2006. 33:424–426.


42. Yang XY, Yang DS, Wei-Zhang , Wang JM, Li CY, Hui-Ye , Lei KF, Chen XF, Shen NH, Jin LQ, Wang JG. 10-hydroxy-2-decenoic acid from royal jelly: A potential medicine for RA. J Ethnopharmacol. 2010. 128:314–321.


43. Izuta H, Chikaraishi Y, Shimazawa M, Mishima S, Hara H. 10-hydroxy-2-decenoic acid, a major fatty acid from royal jelly, inhibits VEGF-induced angiogenesis in human umbilical vein endothelial cells. Evid Based Complement Alternat Med. 2009. 6:489–494.


44. Fujiwara S, Imai J, Fujiwara M, Yaeshima T, Kawashima T, Kobayashi K. A potent antibacterial protein in royal jelly. purification and determination of the primary structure of royalisin. J Biol Chem. 1990. 265:11333–11337.


45. Kimura M, Kimura Y, Tsumura K, Okihara K, Sugimoto H, Yamada H, Yonekura M. 350-kDa royal jelly glycoprotein (apisin), which stimulates proliferation of human monocytes, bears the beta1-3galactosylated N-glycan: Analysis of the N-glycosylation site. Biosci Biotechnol Biochem. 2003. 67:2055–2058.


46. Koya-Miyata S, Okamoto I, Ushio S, Iwaki K, Ikeda M, Kurimoto M. Identification of a collagen production-promoting factor from an extract of royal jelly and its possible mechanism. Biosci Biotechnol Biochem. 2004. 68:767–773.


47. Blum MS, Novak AF, Taber S. 10-hydroxy-delta 2-decanoic acid, an antibiotic found in royal jelly. Science. 1959. 130:452–453.


48. Townsend GF, Morgan JF, Hazlett B. Activity of 10-hydroxydecanoic acid from royal jelly against experimental leukaemia and ascitic tumours. Nature. 1959. 183:1270–1271.


49. Varga J, Jimenez SA. Stimulation of normal human fibroblast collagen production and processing by transforming growth factor-β. Biochem Biophys Res Commun. 1986. 138:974–980.


50. Echigo T, Takenaka T, Yatsunami K. Comparative studies on chemical composition of honey, royal jelly and pollen loads. Bull Fac Agr Tamagawa Univ. 1986. 26:1–26.