Abstract
A change in pH can alter the intracellular concentration of electrolytes such as intracellular Ca2+ and Na+ ([Na+]i) that are important for the cardiac function. For the determination of the role of pH in the cardiac magnesium homeostasis, the intracellular Mg2+ concentration ([Mg2+]i), membrane potential and contraction in the papillary muscle of guinea pigs using ion-selective electrodes changing extracellular pH ([pH]o) or intracellular pH ([pH]i) were measured in this study. A high CO2-induced low [pH]o causes a significant increase in the [Mg2+]i and [Na+]i, which was accompanied by a decrease in the membrane potential and twitch force. The high [pH]o had the opposite effect. These effects were reversible in both the beating and quiescent muscles. The low [pH]o-induced increase in [Mg2+]i occurred in the absence of [Mg2+]o. The [Mg2+]i was increased by the low [pH]i induced by propionate. The [Mg2+]i was increased by the low [pH]i induced by NH4Cl-prepulse and decreased by the recovery of [pH]i induced by the removal of NH4Cl. These results suggest that the pH can modulate [Mg2+]i with a reverse relationship in heart, probably by affecting the intracellular Mg2+ homeostasis, but not by Mg2+ transport across the sarcolemma.
Although the biophysiological importance of magnesium has long been recognized [28] since it was first demonstrated to play an essential role in mammals 75 years ago [19], the mechanism for how magnesium is transported across the cell membrane and how the cells regulate its intracellular level is unclear. Nowadays, a magnesium deficiency is believed to be a major contributory factor to many diseases and the role of magnesium as a therapeutic agent has been tested in many large clinical trials [28]. The problem associated with the use of magnesium ion (Mg2+) in a clinical setting is the fundamental lack of understanding of Mg2+ transport and homeostasis [27].
In the mammalian heart, there should be several processes that act in concert to maintain the intracellular Mg2+ concentration ([Mg2+]i) constant below 1 mM in the bulk cytosol because the total Mg2+ concentration has been reported to be up to 25 mM [23]. These processes include membrane transport systems and intracellular Mg2+ buffering systems including uptake/release from subcellular organelles [8].
Changes in intracellular pH ([pH]i) have been shown to occur in many cell types under various pathophysiological conditions [17]. Therefore, changes in [pH]i may affect the cytosolic electrolyte concentrations including changes in the intracellular Ca2+ concentration ([Ca2+]i) [12,24], Na+ concentration ([Na+]i) [5,13] and possibly [Mg2+]i, which can cause various pathophysiological conditions.
An increase in the [Mg2+]i evoked by intracellular acidosis has been reported in isolated chicken heart cells [9], rat cardiomyocytes [20] and cortical neurons [26], while no change was reported in ferret ventricular muscles [6] and a decrease in amnion cells [22] and leech Retzius neurons [11]. Although few studies at the cellular level have addressed the role of [pH]i in cardiac Mg2+ homeostasis, its role at the tissue level is unclear and particularly controversial.
Therefore, the aim of this study was to examine the role of pH in cardiac Mg2+ regulation by the simultaneous measurements of the [Mg2+]i, membrane potential (Em) and twitch force (TF) in papillary muscle of guinea pig using ion-selective electrodes changing the extracellular pH ([pH]o) or [pH]i.
The papillary muscles (2~3 mm long, ~0.5 mm diameter) were isolated from the right ventricle of guinea pigs (either gender weighing 250~350 g, Bio-Safety Research Institute of Chonbuk National University) according to the Guide for the Care and Use of Laboratory Animals (ILAR, USA). Mounted in a superfusion chamber, the muscle was stimulated by 1 Hz rectangular pulses with a 1 msec duration at 1.2 times the threshold voltage using an electronic stimulator (Narco Biosystem, USA). The isometric tension that developed in the preparation was measured using a force transducer (Cambridge Technology, USA) and recorded on a physiograph (Gould, USA).
The muscle preparation was superfused continuously at 50 ± 2 ml/10 min with a Tyrode solution containing (in mM): NaCl 137, KCl 5.4, CaCl2 1.8, MgCl2 1.1, NaH2PO4 0.45, glucose 5, NaHCO3 11.9; the pH was adjusted to 7.4 ± 0.05 by aeration with 95 % O2 and 5 % CO2 and the temperature was maintained at 38 ± 0.5℃. The [pH]o of the Thyroid solution was altered by modifying the percentage of CO2 in the air mixture. No MgCl2 was added to the nominally Mg2+-free solution. The contaminative magnesium concentration of the Mg2+-free solution (nominally free of extracellular Mg2+) was less than 0.9 nM using atomic absorption spectroscopy (Analab, Korea). The NaCl in the Tyrode solution was replaced with Na+-propionate on an equimolar basis. For the experiments controlling the NH4Cl concentration, an equimolar amount of choline chloride was added to the Thyroid solution.
Beveling, silanization and calibration of microelectrode was carried out with a slight modification of the methods reported elsewhere [3,6,16] Briefly, the microelectrodes were pulled from borocilicate glass capillaries (Richland, USA and World Precision, USA) using a vertical puller (David Kopf, USA). Conventional microelectrodes were used as the internal reference electrodes and the electrical resistance was 20~30 MW when filled with 3 M KCl. The tributylchlorosilane-silanized microelectrodes were backfilled with 100 mM NaCl for the Na+-selective microelectrodes and 100 mM MgCl2 for the Mg2+-selective microelectrodes, and then beveled to increase the tip diameter to ~1 mm. The microelectrodes were filled with the Na+ neutral carrier, ETH 227, and the Mg2+ neutral carrier, ETH 5214, respectively. Calibration was carried out with a fixed ionic background to mimic intracellular conditions and thereby to minimize effects of interfering ions (unorthodox calibration). The possibility of the interference to Mg2+-selective electrodes by H+, Na+ was excluded by the use of ETH 5214 [3]. The Mg2+-selective electrodes used in these experiments had 0.15 mM of detection limit and more than 52 mV of the slope between 10 and 0.1 mM MgCl2. The Na+-selective electrodes used in these experiments had more than 61 mV of the slope between 100 and 1 mM NaCl [16]. As the electrodes did not behave linearly in the physiological range, their calibration curves were fitted with the Nicolsky-Eiseman equation. Electrodes were only used when their slope in the linear range of the electrode was at least 90% of the Nernstian slope. After every successful experiment, the electrodes were recalibrated. While the two calibration curves for Na+ electrodes were usually in good agreement, most Mg2+ electrodes showed a considerable loss in sensitivity during an experiment. An experiment was used for quantitative evaluation when the values calculated from the two calibration curves did not differ by more than 0.4 mM [6].
The ion-selective and conventional electrodes were inserted into the beating papillary muscle within a 1 mm distance. The intracellular membrane potential (Em) was recorded using a conventional electrode and the intracellular potential (ENa or EMg) was recorded by each of the ion-selective electrodes. These electrodes were referenced to the potential of a reference electrode placed in a superfusing solution close to the impaling sites. The potential sensitivity to intracellular Na+ (ENa-Em) and Mg2+ (EMg-Em) was converted to [Na+]i and [Mg2+]i, respectively, using an individual calibration curve for each electrode.
The NaCl, KCl, CaCl2, MgCl2, NaH2PO4, glucose, NaHCO3, choline chloride, Na+-propionate and NH4Cl were purchased from the Sigma-Aldrich (USA). The N-tributyl-chlorosilane, ETH 5214 (Magnesium ionophore II-cocktail A), ETH 227 (Sodium ionophore I-cocktail A), Mg2+ stock and Na+ stock solutions were purchased from Fluka (Switzerland). All the chemicals were prepared as concentrated stock solutions and diluted with the Tyrode solution or a proper solvent. The final solvent concentration in experimental solutions did not exceed 0.1%.
The results are presented as a mean ± SD. The data was analyzed using a Student's t-test and repeated measures analyses of variance, one-way ANOVA. A p value less than 0.05 was considered significant.
In the papillary muscles, lowering the [pH]o, which was achieved by increasing the partial pressure of CO2, had a negative inotropic effect, and depolarization of Em. In addition, it evoked a significant increase in the [Mg2+]i and [Na+]i. Figs. 1 and 2 show a typical experimental result. In 22 beating muscles, the extracellular acidosis from pH 7.4 to 6.4 induced depolarization of the Em by 5.5 ± 1.8 mV from the control value of 83.3 ± 2.6 mV, and decreased the TF by 19.0 ± 3.5% of control level over a 5 min period. It also led to a definite reversible increase in the [Mg2+]i by 0.36 ± 0.03 mM (n = 22) and an enormous increase in [Na+]i by 2.36 ± 0.12 mM (n = 6). There were no significant differences between the beating and quiescent states in these results.
Increasing the [pH]o had opposite effects as illustrated in Fig. 1. During 10 min, the extracellular alkalosis from the pH 7.4 to 8.4 slightly decreased the [Mg2+]i by 0.12 ± 0.03 mM (n = 12), hyperpolarized the Em by 1.3 ± 0.5 mV and increased the TF by 257 ± 22.4%.
The [pH]o was altered in an absence of [Mg2+]o in order to determine if the change in the [Mg2+]i caused by the extracellular acidification was due to Mg2+ influx from the extracellular Mg2+. As shown in Fig. 3, the low [pH]o-induced increase in the [Mg2+]i in the absence of [Mg2+]o was similar to that in the presence of a [Mg2+]o. In addition, the low [pH]o-induced increase in the [Mg2+]i in 20 mM [Mg2+]o was similar to that in 1.1 mM [Mg2+]o (data not shown.).
In order to determine if a change in [pH]i can alter [Mg2+]i, the [pH]i was manipulated at a constant [pH]o and the subsequent effect on the [Mg2+]i in the heart was examined. As shown in Fig. 4, 20 mM propionate, which elicited intracellular acidosis in the cardiac myocytes [6,18], caused a rapid increase in the [Mg2+]i (0.16 ± 0.03 mM, n = 6) at a constant [pH]o of 7.4. After the muscle was superfused with the normal Thyroid solution, the [Mg2+]i promptly recovered to the control level. There was a rapid hyperpolarization of the Em (2.8 ± 0.4 mV) followed by a slower partial recovery with the treatment with propionate. After withdrawing the propionate, the Em rapidly depolarized (1.7 ± 0.3 mV) and slowly recovered. The TF was increased slightly by propionate but not significantly. After withdrawing the propionate, the TF was enforced twofold (196.8 ± 13.3% of control).
Perfusion with 20 mM NH4Cl causes transient intracellular alkalinization, and the removal of NH4Cl elicits transient intracellular acidification [9,17]. Fig. 5 shows the time course of the changes in the [Mg2+]i, Em and TF evoked by NH4Cl in the papillary muscle. During perfusion with 20 mM NH4Cl, there were a sustained increase in [Mg2+]i (0.23 ± 0.05 mM, n = 6), enormous depolarization (11.0 ± 1.0 mV) and a transient increase (146.8 ± 11.3% of control) in the TF following a significant sustained decrease (36.7 ± 5.4% of control). After removing the NH4Cl, the [Mg2+]i increased transiently and then recovered to the control level accompanied by a transient increase in the TF, which was below the control level.
Magnesium is the 4th most common mineral salt in vertebrates after phosphorus, calcium, and potassium. Mg2+ is also the 2nd most common intracellular ion after K+ and the 4th most common plasma ion after Na+, K+ and Ca2+ [21]. It is an essential cofactor in the activation of more than 320 enzyme systems in many organisms [21], involved in carbohydrates, lipid, proteins and the DNA metabolism, interacting either with the substrate or with the enzyme directly. Despite the abundance of Mg2+ within all cells and its importance in animal life, its general roles in the cellular function are not well understood for a variety of reasons [23].
It has been reported that submillimolar [Mg2+]i significantly influence many intracellular processes in the cardiac muscles, including the adenylate cyclase activity [21], Na+ pathways [6], K+ pathways [1], excitation-contraction coupling [12], Ca2+ sensitivity of myofilaments [2] and Ca2+ binding to intracellular sites [14]. [Mg2+]i is maintained in a relatively narrow concentration range to ensure proper functioning of the cells.
Intracellular pH is an important modulator of cardiac function, influencing processes as varied as contraction [4,13], excitation [30] and electrical arrhythmia [25]. Protons are produced metabolically within the heart, they are highly reactive with cellular proteins, and they must be removed if cardiac function is to be maintained. A sophisticated system for regulating pHi has therefore evolved in heart cells. Steady-state pHi is typically 7.1-7.3 [17]. It can decline modestly with an increase in heart rate [4,7] and, more dramatically, during myocardial ischemia [10]. Although few studies at the cellular level have addressed the role of [pH]i in cardiac Mg2+ homeostasis, its role at the tissue level is unclear and particularly controversial.
This study found that extracellular acidification led to a definite reversible increase in [Mg2+]i in the papillary muscles from guinea pigs. The high [pH]o had opposite effect. The low [pH]i-induced increase in [Mg2+]i might be induced by an increase in Mg2+ influx or the modulation of the intracellular Mg2+ homeostasis. The extracellular acidification also caused an huge increase in [Na+]i in the papillary muscles. This might result in a decrease in the driving force for the Na+/Mg2+ exchange and cause the decrease in [Mg2+]i. An acidification-induced increase in [Mg2+]i was also observed whilst exposing the muscles to a nominally Mg2+-free bath solution. In addition, the low [pH]o-induced increase in the [Mg2+]i in 20 mM [Mg2+]o was similar to that in 1.1 mM [Mg2+]o. These results suggest that acidification did not cause Mg2+ influx such as transport through Na+/Mg2+ exchange but possibly a redistribution of the intracellularly bound Mg2+. Such redistribution might involve the Mg2+ binding within the cytoplasm or the Mg2+ storing in organelles [8].
If the extracellular acidification directly caused Mg2+ influx, Mg2+/H+ exchange and/or Mg2+/HCO3- cotransport would be a strong candidate for involvement in the influx. If Mg2+/H+ exchange were present in the cardiac sarcolemma as in the epithelium [18], extracellular acidification would increase its driving force and cause a decrease rather than an increase in [Mg2+]i until the [pH]i is reduced to electrochemical equivalent level of [pH]o. However, the low [pH]o immediately caused an increase in [Mg2+]i. If the exchange worked as a H+-exporting and Mg2+-importing mechanism, it should have caused an increase in [Mg2+]i during [pH]i recovery from pH 6.4 to 7.4 when reperfusing. However, a decrease in [Mg2+]i was observed during [pH]i recovery. Therefore, Mg2+/H+ exchange plays no role in the acidification-induced increase in [Mg2+]i. Nevertheless, the acidification-induced modulation in the [Mg2+]i coupled with HCO3- such as Mg2+/HCO3- cotransport in sarcolemma and mitochondrial membrane [29] and with H+ such as Mg2+/H+ exchange in the mitochondrial membrane cannot be excluded [15].
It is well known that a high CO2-induced extracellular acidification can produce a low [pH]i [4]. The decrease in [pH]i results from the intracellular hydration of CO2 and the subsequent dissociation to produce H+ and HCO3-. Changing the [pH]o from 6.4 to 8.4 caused a linear change in [pH]i in same direction of 0.085 [pH]i units/[pH]o units in ferret ventricular muscle [3]. In the papillary muscle of guinea pigs, the [pH]o was reduced from 7.4 to 6.5, and [pH]i would be expected to decrease by only 20~40% of the [pH]o change [4]. Therefore, it is possible that the extracellular acidification increased the [Mg2+]i, which is dependent upon a decrease in [pH]i.
In order to clarify this possibility, the changes in [pH]i while maintaining a constant [pH]o of 7.4 were examined. Intracellular acidification by Na+-propionate results from the influx of uncharged propionate and the subsequent intracellular dissociation [17]. Intracellular acidification can occur even when the [pH]o is neutral, because only the concentration gradient of the neutral form is the driving force. In this study, the [Mg2+]i in the papillary muscle was increased as a result of propionate exposure, which can cause a low [pH]i at neutral [pH]o. The addition and removal of NH4Cl can lead to large transient changes in the [pH]i [9,17]. There are four phases to this process: 1) phase 1; rapid alkalinization by addition, 2) phase 2; slow acidification, 3) phase 3; rapid acidification by removal and 4) phase 4; recovery of [pH]i. This study found that the [Mg2+]i was increased by the subsequent acidification (phase 2) by NH4Cl in the papillary muscle, which was enforced by the rapid acidification (phase 3) and recovered to the control level by the recovery of [pH]i (phase 4). Because the [Mg2+]i had not been affected by the intracellular alkalinization (phase 1), the size and time of alkalinization could not sufficiently alter the [Mg2+]i. Indeed, the effect of alkalinization on the [Mg2+]i was lower than that of acidification. Therefore, changes in [pH]i can affect the [Mg2+]i in the heart; acidification increases the [Mg2+]i and alkalinization decreases the [Mg2+]i. Similar conclusions were also reported by Feudenrich et al. [9], who used Mag-fura 2 AM to determine the [Mg2+]i of single chick cardiomyocytes, and by Li and Quamme [20], who examined isolated rat cardiomyocytes. Feudenrich et al. showed acidification (0.89 pH units) with the use of 10 mM NH4Cl increases the [Mg2+]i from the basal levels of 0.35 to 0.47 mM. Alkalinization of the heart cells from pH 7.11 to 8.32 modestly decreased the Mg2+ activity by 0.02 mM. Li and Quamme reported that the [Mg2+]i within a single cell decreased by 129 ± 13 mM with rapid alkalinization from the basal levels of pH 7.1 to 7.6 following a NH4+ pulse. The removal of the NH4Cl bathing solution caused cytosolic acidification, pH 6.9, and an increase in the [Mg2+]i, from 467 ± 47 to 569 ± 41 mM. Rajdev and Reynolds [26] induced intracellular acidification of 2.46 pH units by the withdrawal of 25 mM NH4Cl and observed a mean increase in the [Mg2+]i of 0.62 mM in the cortical neurons. Therefore, the pH can regulate the [Mg2+]i with a reverse relationship at tissue level as well as at cell level in the heart.
In conclusion, pH could regulate the [Mg2+]i in guinea pig heart; acidification increases the [Mg2+]i and alkalinization decreases the [Mg2+]i. The modulation of [Mg2+]i might be the result of a change in the intracellular Mg2+ buffering, but not by the transportation of Mg2+ across the sarcolemma.
Figures and Tables
Fig. 1
Effects of changes in [pH]o on [Mg2+]i in papillary muscle. Typical recordings of the membrane potential (Em), intracellular Mg2+ concentration ([Mg2+]i) and twitch force (TF) during the change in extracellular pH ([pH]o) in the beating state. Extracellular acidification was induced by regulating the CO2/O2 composition.
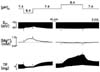
Fig. 2
Effects of low [pH]o on the [Na+]i. Typical recordings of the Em, [Na+]i and TF during a change in [pH]o in the beating state. Extracellular acidification was induced by regulating the CO2/O2 composition.
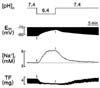
Fig. 3
Effects of [Mg2+]o on low [pH]o-induced increase in [Mg2+]i. Typical recordings of the Em and [Mg2+]i during a change in [pH]o in the absence and presence of [Mg2+]o in a quiescent muscle. Extracellular acidification was induced by regulating the CO2/O2 composition. No MgCl2 was added to the Mg2+-free solution (nominally absence of extracellular Mg2+).
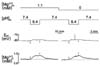
Acknowledgments
This work was supported by a grant from the Korea Science and Engineering Foundation (08-2003-000-10605-0) and the Regional Research Centers Program of the Korean Ministry of Education & Human Resources Development through the Center for Healthcare Technology Development.
References
1. Agus ZS, Morad M. Modulation of cardiac ion channels by magnesium. Annu Rev Physiol. 1991. 53:299–307.


2. Aomine M, Tatsukawa Y, Yamato T, Yamasaki S. Antiarrhythmic effects of magnesium on rat papillary muscle and guinea pig ventricular myocytes. Gen Pharmacol. 1999. 32:107–114.


3. Blatter LA, McGuigan JA. Intracellular pH regulation in ferret ventricular muscle. The role of Na-H exchange and the influence of metabolic substrates. Circ Res. 1991. 68:150–161.


4. Bountra C, Vaughan-Jones RD. Effect of intracellular and extracellular pH on contraction in isolated, mammalian cardiac muscle. J Physiol. 1989. 418:163–187.


5. Borle AB, Bender C. Effects of pH on Ca2+i, Na+i, and pHi of MDCK cells: Na(+)-Ca2+ and Na(+)-H+ antiporter interactions. Am J Physiol. 1991. 261:C482–C489.


6. Buri A, Chen S, Fry CH, Illner H, Kickenwiez E, McGuigan JAS, Noble D, Powell T, Twist VW. The regulation of intracellular Mg2+ in guinea-pig heart, studied with Mg2+-selective microelectrodes and fluorochromes. Exp Physiol. 1993. 78:221–233.


7. Elliott AC, Smith GL, Allen DG. The metabolic consequences of an increase in the frequency of stimulation in isolated ferret hearts. J Physiol. 1994. 474:147–159.


8. Flatman PW. Mechanism of magnesium transport. Annu Rev Physiol. 1991. 53:259–271.
9. Freudenrich CC, Murphy E, Levy LA, London RE, Lieberman M. Intracellular pH modulates cytosolic free magnesium in cultured chicken heart cells. Am J Physiol. 1992. 262:C1024–C1030.


10. Garlick PB, Radda GK, Seeley PJ. Studies of acidosis in the ischaemic heart by phosphorus nuclear magnetic resonance. Biochem J. 1979. 184:547–554.


11. Gunzel D, Durry S, Schlue WR. Intracellular alkalinization causes Mg2+ release from intracellular binding sites in leech Retzius neurons. Pflugers Arch. 1997. 435:65–73.
12. Hall SK, Fry CH. Magnesium affects excitation, conduction, and contraction of isolated mammalian cardiac muscle. Am J Physiol. 1992. 263:H622–H633.


13. Harrison SM, Frampton JE, McCall E, Boyett MR, Orchard CH. Contraction and intracellular Ca2+, Na+ and H+ during acidosis in rat ventricular myocytes. Am J Physiol. 1992. 262:C348–C357.
14. Iseri LT, French JH. Magnesium: nature's physiologic calcium blocker. Am Heart J. 1984. 108:188–193.


15. Jung DW, Brierley GP. Magnesium transport by mitochondria. J Bioenerg Biomembr. 1994. 26:527–535.


16. Lee CO, Im WB, Sonn JK. Intracellular sodium ion activity: reliable measurement and stimulation-induced change in cardiac Purkinje fibers. Can J Physiol Pharmacol. 1987. 65:954–962.


17. Leem CH, Lagadic-Gossmann D, Vaughan-Jones RD. Characterization of intracellular pH regulation in the guinea-pig ventricular myocyte. J Physiol. 1999. 517:159–180.


18. Leonhard-Marek S, Gabel G, Martens H. Effects of short chain fatty acids and carbon dioxide on magnesium transport across sheep rumen epithelium. Exp Physiol. 1998. 83:155–164.


19. Leroy J. Nécessité du magnésium pour la croissence da la souris. omptes Rendus des Sceances de la Société de Biologie. 1926. 94:341.
20. Li HY, Quamme GA. Effect of pH on intracellular free Mg2+ in isolated adult rat cardiomyocytes. Biochim Biophys Acta. 1994. 1222:164–170.


21. Maguire ME, Cowan JA. Magnesium chemistry and biochemistry. BioMetals. 2002. 15:203–210.
22. Masumoto N, Tasaka K, Mizuki J, Miyake A, Tanizawa O. Regulation of intracellular Mg2+ by superoxide in amnion cells. Biochem Biophys Res Commun. 1992. 182:906–912.
23. McGuigan JAS, Elder HY, Gunzel D, Schlue W-R. Magnesium Homeostasis in Heart; A Critical Reappraisal. J Clin Basic Cardiol. 2002. 5:5–22.
24. Negulescu PA, Machen TE. Lowering extracellular sodium or pH raises intracellular calcium in gastric cells. J Membr Biol. 1990. 116:239–248.


25. Orchard CH, Cingolani HE. Acidosis and arrhythmias in cardiac muscle. Cardiovas Res. 1994. 28:1312–1319.


26. Rajdev S, Reynolds IJ. Calcium influx but not pH or ATP level mediates glutamate induced changes in intracellular magnesium in cortical neurons. J Neurophysiol. 1995. 74:942–949.


27. Romani AM, Maguire ME. Hormonal regulation of Mg2+ transport and homeostasis in eukaryotic cells. Biometals. 2002. 15:271–283.
28. Saris NE, Mervaala E, Karppanen H, Khawaja JA, Lewenstam A. Magnesium. An update on physiological, clinical and analytical aspects. Clin Chim Acta. 2000. 294:1–26.
29. Schweigel M, Vormann J, Martens H. Mechanisms of Mg(2+) transport in cultured ruminal epithelial cells. Am J Physiol Gastrointest Liver Physiol. 2000. 278:G400–G408.