Abstract
We studied the expression of caveolin-1 in the spinal cords of rats using 60Co γ-ray irradiation (single dose of 8 Gray (Gy)) in order to determine the possible involvement of caveolin-1 in the tissues of the central nervous system after irradiation. Spinal cords sampled at days 1, 4, and 9 post-irradiation (PI) (n = 5 per each time point) were analyzed by Western blot and immunohistochemistry. Western blot analysis showed that the expression of caveolin-1 was significantly increased at day 1 PI (p < 0.05), and returned to the level of normal control rats on days 4 and 9 PI. Immunohistochemistry showed that caveolin-1 immunoreactivity was enhanced in some glial cells, vascular endothelial cells, and neurons in the spinal cords. The increased expression of glial fibrillary acidic protein (GFAP), a marker for an astroglial reaction, was consistent with that of caveolin-1. In addition, caveolin-1 was co-localized in hypertrophied GFAP-positive astrocytes. Taking all these facts into consideration, we postulate that irradiation induces the increased expression of caveolin-1 in cells of the central nervous system, and that its increased expression in astrocytes may contribute to hypertrophy of astrocytes in the spinal cord after irradiation. The precise role of caveolin-1 in the spinal cords should be studied further.
Radiation has been widely used to treat common central nervous system (CNS) cancers, particularly glioblastoma multiforme, with or without chemotherapy [2,6] and for supportive treatment of spinal cord injury in animals [23]. Although CNS tissues are considered radioresistant [18], radiation induces increased permeability of the blood-brain barrier, edema in the early stages of therapy [12], astrogliosis in the later stages of therapy [10], and apoptosis and proliferation of glial cells [1,10,22]. Furthermore, it is known that radiation increases the expression of intercellular adhesion molecule-1 in astrocytes, which facilitate the inflammatory process [13]. It was recently demonstrated that radiation-induced activation of microglia stimulates astrogliosis in the rat brain, indicating the increased expression of glial fibrillary acidic protein (GFAP) [8].
Caveolins are oligomeric proteins of 22-24 kDa, and present in plasma cell membrane, mitochondria, endoplasmic reticulum, the Golgi/trans-Golgi network, and secretory vesicles. They play a critical role in normal vesicular transport, cholesterol homeostasis, and signal transduction, and are also associated with several human diseases, including multiple myeloma [7,15,19,21].
During the pathological process in the CNS after irradiation, it is possible that a variety of lipid raft proteins, including caveolin-1, is activated; these proteins subsequently mediate intracellular signaling. Caveolin-1 is the principal structural and functional component of caveolae, a plasmalemmal compartment that has been proposed to sequester lipid and protein components that participate in a variety of cellular functions, including signal transduction, lipid metabolism, cell proliferation, and apoptotic cell death [4,9,14,20].
Although many death-related signals have been studied in the CNS tissues after irradiation, the changes of the lipid raft proteins, including caveolin-1, after irradiation have not been clarified in the CNS, particularly in the spinal cord. The goal of the present study was to evaluate whether the expression of caveolin-1, a lipid raft protein, was affected after irradiation in the CNS, particularly in the spinal cord, with special reference to the hypertrophy of astrocytes.
Sprague-Dawley rats were purchased from Daehan Biolink (Korea) and bred in our animal facility. Five- to six-week-old male rats weighing 121.1 ± 19.2 g were used throughout the experiments. The animals were housed in cages in a standard barrier facility and maintained on a 12-h light:12-h dark cycle at 23℃. The research was conducted in accordance with the internationally accepted principles for laboratory animal use and care, as found in the NIH guidelines (USA).
Irradiation was carried out using a 60Co γ-ray source (370 TBq; Co-60 Irradiation Facility, Applied Radiological Science Research Institute, Cheju National University, Korea). Animals were anesthetized with chloral hydrate (375 mg/kg body weight, peritoneal injection), and the whole bodies of the rats were irradiated, each with a single dose of 8 Gray (Gy). Groups were subsequently killed on days 1, 4, and 9 PI (n = 5 per each time point), respectively. Control animals were not irradiated.
In order to study the expression of caveolin-1 in the spinal cords, tissues were sampled on days 0 (control), 1, 4, and 9 PI (n = 5 per each time point). The spinal cords were removed and frozen at -70℃ for protein analysis. Some pieces of spinal cords were fixed in 4% paraformaldehyde in phosphate-buffered saline (PBS) at pH 7.4. Specimens were processed using standard paraffin embedding methods, and were cut to a thickness of 5 µm for routine hematoxylin-eosin staining and immunohistochemical studies.
The specificity of rabbit polyclonal anti-caveolin-1 (N-20) (Santa Cruz Biotechnologies, USA) has been well characterized by the manufacturer. The N-20 was an affinity-purified rabbit antibody raised against a peptide mapping at the N-terminus of caveolin-1 of human origin according to the data sheet supplied by the manufacturer. Mouse monoclonal anti-GFAP (Sigma, USA) was used for the detection of astrocytes. Mouse monoclonal anti-beta actin was also obtained from Sigma (USA).
Spinal cords were homogenized in lysis buffer (40 mM Tris, 120 mM NaCl, 0.1% Nonidet P-40, 2 mM Na3VO4, 1 mM phenylmethylsulfonyl fluoride, 10 µg/ml aprotinin, 10 µg/ml leupeptin) with 20 strokes in a homogenizer. The homogenates were transferred into microtubes and centrifuged at 12,000 rpm for 20 min; the supernatant was then harvested.
For the immunoblot assay, supernatant containing 20 µg of protein was loaded into each lane of a 10% SDS-PAGE gel and transferred onto a nitrocellulose membrane (Schleicher & Schuell BioScience, USA). The residual binding sites on the membrane were blocked by incubation with 5% nonfat milk in Tris-buffered saline (TBS; 10 mM Tris-HCl [pH 7.4] and 150 mM NaCl) for 1 h and then incubated with each primary antibody, including anti-caveolin-1 (1 : 1000) and anti-GFAP (1 : 20,000), for 2 h. The blots were washed three times in TBS containing 0.1% Tween-20, and were then probed with appropriate secondary antibodies including horseradish peroxidase-conjugated anti-rabbit IgG or anti-mouse IgG (Vector, USA) for 1 h. The blots were developed using enhanced chemiluminescence (ECL) reagents according to the instructions of the manufacturer. After visualization with ECL, the antibodies were stripped from the membranes, which were reprobed with monoclonal anti-beta actin antibody (Sigma, USA). The density of each band obtained by Western blot analysis was measured with a scanning laser densitometer (GS-700; Bio-Rad, USA) and analyzed using Molecular Analyst software (Bio-Rad, USA). The ratios of caveolin-1/beta-actin were compared. The results were analyzed statistically by one-way ANOVA followed by the Newman-Keuls test. In all cases, p < 0.05 was considered significant.
After deparaffinization and hydration, the sections were treated with 0.3% hydrogen peroxide in deionized water for 20 min to block endogenous peroxidase. Some paraffin sections for anti-caveolin-1 immunostaining were boiled in 10 mmol/L sodium citrate (pH 6.0) for 10 min at 95℃. After three washes with PBS, the sections were exposed to 10% normal goat serum and then incubated with each primary antiserum including rabbit polyclonal anti-caveolin-1 (1 : 200) for 1 h at room temperature. After three washes, the appropriate biotinylated secondary antibody and the avidin-biotin-peroxidase complex (ABC) from the Elite kit (Vector, USA) were added sequentially. Peroxidase was developed with a diaminobenzidine tetrahydrochloride (DAB) substrate kit (Vector, USA). The sections were counterstained with hematoxylin prior to mounting.
For double-staining of two antigens in the same section, double-immunofluorescence was applied using fluorescein isothiocyanate (FITC)-labeled goat anti-rabbit IgG (1 : 50 dilution; Sigma, USA) and tetramethyl rhodamine isothiocyanate (TRITC)-labeled goat anti-mouse IgG (1 : 50 dilution; Sigma, USA) secondary antibodies in order to co-localize caveolin-1 and GFAP (1 : 800) in the same cell.
Western blot analysis of caveolin-1 showed that the expression of caveolin-1 significantly increased (fold increase [mean ± SE]: 4.1 ± 1.9, p < 0.05) at day 1 PI compared to those of normal spinal cords, and returned to the level of normal controls at days 4 and 9 PI (Fig. 1). This finding suggests that caveolin-1 is constitutively expressed in the normal spinal cord of the rat, and that it transiently increases in the spinal cord following irradiation.
Western blot analysis of GFAP expression in the spinal cords of normal and irradiated rats is shown in Fig. 2. GFAP immunoreactivity was significantly increased at day 1 PI (1.51 ± 0.06, p < 0.05) and day 4 PI (1.99 ± 0.2, p < 0.01) compared to those of normal spinal cords, and declined thereafter to the normal level at 9 PI (Fig. 2). This finding suggests that irradiation stimulates the expression of GFAP in the spinal cords of rats.
Immunohistochemical analysis was applied to visualize the cell phenotype expressing caveolin-1 in the spinal cords of normal control and irradiated rats. In the normal control rats, caveolin-1 was weakly immunostained in some vascular endothelial cells, neurons, and some glial cells (Fig. 3A&C). The immunoreactivity of caveolin-1 was intense in some vascular endothelial cells, neurons, and glial cells from the spinal cords of irradiated rats at day 1 PI (Fig. 3B&D). These findings indicate that various CNS cells, including glial cells, are responsive to irradiation with a concomitant increase of caveolin-1.
A double-labeling experiment was performed to identify the cell phenotype expressing caveolin-1, and to determine whether hypertrophied astrocytes contain enhanced levels of caveolin-1. This is based on the knowledge that astrogliosis is a prominent feature of radiation injury. Using double-immunofluorescence, both caveolin-1- (Fig. 4A) and GFAP- (Fig. 4B) positive astrocytes were rarely observed in the normal spinal cords (Fig. 4C), while the number of caveolin-1- (Fig. 4D) and GFAP- (Fig. 4E) positive cells was more abundant at day 1 PI (Fig. 4F). These findings suggest that the expression of caveolin-1 is increased in hypertrophied astrocytes in the spinal cord after irradiation.
This is the first study to confirm the increased expression of caveolin-1 in the spinal cord after irradiation. Based on Western blot analysis of caveolin-1, our results suggest that the expression of caveolin-1 transiently increases in spinal cords at day 1 post-irradiation. Immunohistochemical data was largely matched with those of Western blot analysis. In particular, we have found that constitutively expressed caveolin-1 was enhanced in some glial cells, vascular endothelial cells, and neurons in the spinal cord at day 1 PI.
There is general agreement that caveolin-1 has two contradictory functions, in cell proliferation and cell death [14,17,20]. Although many studies have focused on the suppressive role of caveolin-1 in various cell types, caveolin-1 has also been known to be involved in cell proliferation. In the present study, we only examined the intimate relationship between caveolin-1 and hypertrophied astrocytes. This relationship exists because irradiation has been found to induce the increased expression of GFAP in vivo [8,10], and concomitantly increased the expression of caveolin-1 in the present study. This finding is further supported in highly proliferative glial cell lines [4,17] and hypertrophied astrocytes in the spinal cord with experimental autoimmune encephalomyelitis [16].
Regarding the involvement of astrocyte hypertrophy in the CNS after irradiation, we have indirectly confirmed that GFAP and caveolin-1 are intimately associated in the same cells after irradiation. In line with the same patterns of caveolin-1 and GFAP after irradiation, we postulate that increased expression of caveolin-1 is associated with activation of intermediate filaments of astrocytes leading to astrogliosis in this study.
Vascular endothelial cells contain a number of lipid raft proteins, including caveolin-1. In the present study, we found that caveolin-1 was constitutively expressed in vascular endothelial cells in normal animals, and its expression was enhanced after irradiation. Under the condition of irradiation, it has been reported that endothelial apoptosis initiates the disruption of the acute blood-brain barrier following ionizing radiation [11], resulting in the leakage of plasma into CNS tissues. Based on the previous report, we do not exclude the possibility that caveolin-1 is partially involved in the apoptosis of vascular endothelial cells. Collectively, we only prefer to agree that the role of caveolin-1 in CNS cells depends on the cell types and duration of time after irradiation. Further study is needed to examine whether the caveolin-related astroglial reaction after irradiation is neuroprotective or detrimental in immunologically privileged CNS tissues.
Taking all of these findings into consideration, we postulate that irradiation transiently induces the increased expression of caveolin-1 in a variety of CNS cells, and that caveolin-1 in certain cell types, including astrocytes, may elicit cell activation, leading to astrocyte hypertrophy.
Figures and Tables
Fig. 1
Western blot analysis of caveolin-1 in the spinal cords of normal and irradiated rats. A: Representative photomicrographs of caveolin-1 (upper panel) and β-actin (lower panel) expression in normal and irradiated spinal cords (at days 1, 4, and 9 PI). A single band was seen with the approximate molecular weight of caveolin-1 (22 kDa). The β-actin level is shown in the same blot. B: The bar graph denotes a significant increase in caveolin-1 immunoreactivity in the spinal cord after irradiation at day 1 PI; its expression returned to a normal level at days 4 and 9 PI. Data are the means ± SD of five samples at each time point. *p < 0.05 vs. normal controls.
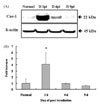
Fig. 2
Western blot analysis of GFAP in normal and irradiated spinal cords. A: Representative photomicrographs of GFAP (upper panel) and β-actin (lower panel) expression in normal and irradiated spinal cords (at days of 1, 4, and 9 PI). B: The bar graph shows a significant increase in GFAP immunoreactivity in the spinal cord after irradiation at days 1 and 4 PI; its expression returned to a normal level at day 9 PI. Data are the means ± SD of five samples at each point. *p < 0.05, **p < 0.01 vs. normal controls.
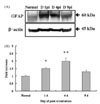
Fig. 3
Immunohistochemical staining of caveolin-1 in the spinal cords of normal control and irradiated rats at day 1 PI. In the normal control spinal cord, caveolin-1 was detected in some vascular endothelial cells (arrowhead), glial cells (A, arrow), and neurons (C, arrowheads). In the spinal cords of rats after irradiation, intense immunostaining of caveolin-1 was detected in some vascular endothelial cells (asterisk), glial cells (B, arrows), and neurons (D, arrowheads) at day 1 PI. A-D: counterstained with hematoxylin. bars = 30 µm.
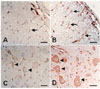
Fig. 4
Double immunofluorescence of caveolin-1 and GFAP in the spinal cords of normal and irradiated rats (day 1 PI). Co-localization of caveolin-1 (A, green, arrow) and GFAP (B, red, arrow) in the normal spinal cords was few (C, merge, arrow), while co-expression of caveolin-1 (D, green, arrows) and GFAP (E, red, arrow) was detected more bundantly at day 1 PI (F, merge, arrows) in the irradiated spinal cords. "V": vascular endothelial cells. bars = 30 µm.
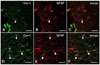
Acknowledgments
This work was supported by a grant from the Research Fund of Cheju National University (2005).
References
1. Atkinson SL, Li YQ, Wong CS. Apoptosis and proliferation of oligodendrocyte progenitor cells in the irradiated rodent spinal cord. Int J Radiat Oncol Biol Phys. 2005. 62:535–544.


2. Burger PC, Vogel FS, Green SB, Strike TA. Glioblastoma multiforme and anaplastic astrocytoma. Pathologic criteria and prognostic implications. Cancer. 1985. 56:1106–1111.


3. Bushby KM. The limb-girdle muscular dystrophies-multiple genes, multiple mechanisms. Hum Mol Genet. 1999. 8:1875–1882.
4. Cameron PL, Liu C, Smart DK, Hantus ST, Fick JR, Cameron RS. Caveolin-1 expression is maintained in rat and human astroglioma cell lines. Glia. 2002. 37:275–290.


5. Colasanti M, Persichini T, Fabrizi C, Cavalieri E, Venturini G, Ascenzi P, Lauro GM, Suzuki H. Expression of a NOS-III-like protein in human astroglial cell culture. Biochem Biophys Res Commun. 1998. 252:552–555.


6. Fisher BJ, Scott C, Macdonald DR, Coughlin C, Curran WJ. Phase I study of topotecan plus cranial radiation for glioblastoma multiforme: results of Radiation Therapy Oncology Group Trial 9507. J Clin Oncol. 2001. 19:1111–1117.


7. Hassan GS, Williams TM, Frank PG, Lisanti MP. Caveolin-1-deficient aortic smooth muscle cells show cell autonomous abnormalities in proliferation, migration, and endothelin-based signal transduction. Am J Physiol Heart Circ Physiol. 2006. 290:H2393–H2401.


8. Hwang SY, Jung JS, Kim TH, Lim SJ, Oh ES, Kim JY, Ji KA, Joe EH, Cho KH, Han IO. Ionizing radiation induces astrocyte gliosis through microglia activation. Neurobiol Dis. 2006. 21:457–467.


9. Ikezu T, Ueda H, Trapp BD, Nishiyama K, Sha JF, Volonte D, Galbiati F, Byrd AL, Bassell G, Serizawa H, Lane WS, Lisanti MP, Okamoto T. Affinity-purification and characterization of caveolins from the brain: differential expression of caveolin-1, -2, and -3 in brain endothelial and astroglial cell types. Brain Res. 1998. 804:177–192.


10. Janeczko K, Pawlinski R, Setkowicz Z, Ziaja M, Soltys Z, Ryszka A. Long-term postnatal effect of prenatal irradiation on the astrocyte proliferative response to brain injury. Brain Res. 1997. 770:237–241.


11. Li YQ, Chen P, Haimovitz-Friedman A, Reilly RM, Wong CS. Endothelial apoptosis initiates acute blood-brain barrier disruption after ionizing radiation. Cancer Res. 2003. 63:5950–5956.
12. Moore AH, Olschowka JA, Williams JP, Paige SL, O'Banion MK. Radiation-induced edema is dependent on cyclooxygenase 2 activity in mouse brain. Radiat Res. 2004. 161:153–160.


13. Nordal RA, Wong CS. Intercellular adhesion molecule-1 and blood-spinal cord barrier disruption in central nervous system radiation injury. J Neuropathol Exp Neurol. 2004. 63:474–483.


14. Okamoto T, Schlegel A, Scherer PE, Lisanti MP. Caveolins, a family of scaffolding proteins for organizing "preassembled signaling complexes" at the plasma membrane. J Biol Chem. 1998. 273:5419–5422.


15. Podar K, Anderson KC. Caveolin-1 as a potential new therapeutic target in multiple myeloma. Cancer Lett. 2006. 233:10–15.


16. Shin T, Kim H, Jin JK, Moon C, Ahn M, Tanuma N, Matsumoto Y. Expression of caveolin-1, -2, and -3 in the spinal cords of Lewis rats with experimental autoimmune encephalomyelitis. J Neuroimmunol. 2005. 165:11–20.


17. Silva WI, Maldonado HM, Velazquez G, Rubio-Davila M, Miranda JD, Aquino E, Mayol N, Cruz-Torres A, Jardon J, Salgado-Villanueva IK. Caveolin isoform expression during differentiation of C6 glioma cells. Int J Dev Neurosci. 2005. 23:599–612.


18. Tofilon PJ, Fike JR. The radioresponse of the central nervous system: a dynamic process. Radiat Res. 2000. 153:357–370.


19. Williams TM, Hassan GS, Li J, Cohen AW, Medina F, Frank PG, Pestell RG, Di Vizio D, Loda M, Lisanti MP. Caveolin-1 promotes tumor progression in an autochthonous mouse model of prostate cancer: genetic ablation of Cav-1 delays advanced prostate tumor development in tramp mice. J Biol Chem. 2005. 280:25134–25145.


20. Williams TM, Lisanti MP. The Caveolin genes: from cell biology to medicine. Ann Med. 2004. 36:584–595.


21. Williams TM, Lisanti MP. Caveolin-1 in oncogenic transformation, cancer, and metastasis. Am J Physiol Cell Physiol. 2005. 288:C494–C506.


22. Yang T, Wu SL, Liang JC, Rao ZR, Ju G. Time-dependent astroglial changes after gamma knife radiosurgery in the rat forebrain. Neurosurgery. 2000. 47:407–415.


23. Zhang SX, Geddes JW, Owens JL, Holmberg EG. X-irradiation reduces lesion scarring at the contusion site of adult rat spinal cord. Histol Histopathol. 2005. 20:519–530.