Abstract
Figures and Tables
Fig. 1
Transverse magnetic resonance imaging over time in a dog with brain infarct. Three days after middle cerebral artery occlusion, infarcts in the right thalamus and left caudate nucleus appear hypointense to isointense on T1-weighted (T1W) images (A) and hyperintense on T2-weighted (T2W) (B) and fluid-attenuated inversion recovery (FLAIR) (C) images. At 8 days post-occlusion, the lesions appear hyperintense (arrowheads) on T1W images (D), with increased intensity and clear margination on T2W (E) and FLAIR (F) images. At 35 days, the lesions are hypointense on T1W (G), hyperintense on T2W (H), and exhibit a hypointense center with peripheral hyperintensity on FLAIR images (I).
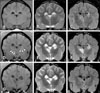
Fig. 2
Tendency in brain infarct intensity over time on T1-weighted magnetic resonance imaging. The lesion appears hypointense during the early stage (e.g., 3 h, 3 days) after middle cerebral artery occlusion (A and E). Lesions show a tendency to appear hypointense at the center with peripheral hyperintensity at 8 days (B and F). The hypointense infarct center has a tendency to expand as the peripheral hyperintensity field narrows (C and G). Most infarcts appear hypointense at 35 days (D and H). The images in panels E, G, and H were acquired from the same dog at post-occlusion days 3, 8, and 35, respectively. The image in panel F was acquired from another dog at 8 days and this lesion appears similar to that seen in panel G at 35 days.
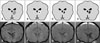
Fig. 3
Diffusion-weighted imaging (DWI) and an apparent diffusion coefficient (ADC) map of a dog with multifocal brain infarcts including the left cerebral lobe. At 8 days after middle cerebral artery occlusion, a lesion locates in the left caudate nucleus and connects with the internal capsule and putamen appears hypointense with peripheral hyperintensity (arrowhead) on DWI (A), and hyperintensity on an ADC map (B). At 35 days, unilateral ventricle enlargement is shown and the lesions appear hypointense with narrowing of the peripheral hyperintense field on DWI (C), and hyperintense on an ADC map (D).
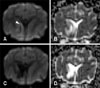
Table 2
Temporal changes in infarcts on magnetic resonance imaging sequences
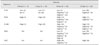
T1W, T1-weighted; T2W, T2-weighted; FLAIR, fluid-attenuated inversion recovery; DWI, diffusion-weighted imaging; ADC, apparent diffusion coefficient; Iso, isointense; NA, not applicable. *A/B indicates relative lesion intensity of peripheral (A) and center (B). †This outcome resulted from T2 shine-through effect.
Table 3
Ventricular size at assessed times after middle cerebral artery occlusion in 11 dogs with infarcts
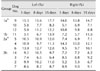
Group 1a, dogs with unilateral cerebrocortical lesions; Group 1b, dogs with bilateral cerebrocortical lesions; Group 2a, dogs having unilateral lesions without cerebrocortical lesions; Group 2b, dogs having bilateral lesions without cerebrocortical lesions. *Groups 1a and 2a had unilateral lesions on the left side.
Acknowledgments
References
















