Abstract
Mesenchymal stem cells (MSCs) have desirable characteristics for use in therapy in animal models and veterinary medicine, due to their capacity of inducing tissue regeneration and immunomodulation. The objective of this study was to evaluate the differences between canine adipose tissue-derived MSCs (AD-MSCs) extracted from subcutaneous (Sc) and visceral (Vs) sites. Surface antigenic markers, in vitro differentiation, and mineralized matrix quantification of AD-MSCs at different passages (P4, P6, and P8) were studied. Immunophenotypic analysis showed that AD-MSCs from both sites were CD44+, CD90+, and CD45−. Moreover, they were able, in vitro, to differentiate into fat, cartilage, and bone. Sc-AD-MSCs preserve in vitro multipotentiality up to P8, but Vs-AD-MSCs only tri-differentiated up to P4. In addition, compared to Vs-AD-MSCs, Sc-AD-MSCs had greater capacity for in vitro mineralized matrix synthesis. In conclusion, Sc-AD-MSCs have advantages over Vs-AD-MSCs, as Sc AD-MSCs preserve multipotentiality during a greater number of passages, have more osteogenic potential, and require less invasive extraction.
Adult-derived mesenchymal stem cells (MSCs) are a non-specialized lineage, with limited ability for auto-renovation and plasticity. Their multipotentiality allows them to differentiate into lineages derived from the same germinal line, mesoderm [530], and also to differentiate into other non-mesodermal lineages [126]. Since a report by Caplan [6], which proposed the use of MSCs in orthopedics, the interest in MSCs has increased due to their potential for use in regenerative medicine. The increased interest is due to the attractive characteristics of MSCs: multipotentiality [11], immunomodulation [219], angiogenesis [1433], homing to the site of inflammation [732], and antimicrobial effect [1320]. All of the aforementioned characteristics indicate the great therapeutic potential of MSCs in human and veterinary medicine. Furthermore, domestic animals have similar pathologies to humans, so they can be used as animal models [31].
In order to be able to compare results between researchers, MSCs used in therapy, must be characterized, following the recommendations of the International Society of Cellular Therapy (ISCT). The MSCs must have a fibroblastic morphology, be adherent to plastic, express mesenchymal stromal markers, and have in vitro tri-differentiation capacity [5]. Different surface markers, such as CD29, CD44, and CD90 have been used to characterize canine adipose tissue-derived MSCs (AD-MSCs) [112430]. Other markers, such as hematopoietic markers CD34 and CD45 need to be absent or have very low expression [1124]. There is limited availability of flow cytometry reagents specific for canines, thus, in most of the cases, human- or mouse-specific antibodies that have cross-reactivity with canines are used. However, one problem is that cross-reactivity results in different proportions of positive cells for a variety of markers; thus, there is controversy over which surface markers are most appropriate for canine MSCs [2224].
Initially, the most common MSC source was bone marrow (BM) [25], but currently, the adipose tissue (AT) source, described for the first time by Zuk et al. [35], is more commonly studied. AD-MSCs have several advantages over BM-derived MSCs, as the use of AT results in more MSCs being obtained and the AT-derived cells have greater proliferative ability [322]. Moreover, the AT extraction method is less invasive [11] and AD-MSCs conserve their undifferentiated state during more passages [15]. These characteristics have motivated researchers to focus on AT as a safe and convenient source of MSCs for use in regenerative medicine [911]. Several studies in humans and animal models have compared the characteristics of AD-MSCs derived from different anatomical sites, such as those in subcutaneous (Sc) and visceral (Vs) areas. Multipotentiality studies on AD-MSCs of both origins have shown contrasting results regarding differentiation capacity and in vitro propagation ability [42122]. There are few in vitro studies comparing immunophenotype and multipotentiality characteristics of canine MSCs derived from different AT sites at different passage numbers. Our hypothesis is that different AT origins and passage numbers have an effect on MSC properties. The objective of the present study was to evaluate, in vitro, differences in immunophenotype and multipotentiality over serial passages of canine AD-MSCs derived from Sc and Vs sites.
The AT samples were extracted from 5 healthy female canines, 5 to 11 months old, in interestrus. Abdominal AT, was extracted from two different sites, flank Sc and periovaric Vs, of canines submitted to ovariectomy. This study was carried out, in strict accordance with the recommendations of the Committee on Ethics of Animal Experiments of Brazil “Comitê de ética no uso de animais do Hospital de Clínicas de Porto Alegre (CEUA/HCPA)” under approval number 130510.
Canines were anesthetized with intravenous propofol (Eurofarma Laboratórios, Brazil; 6 mg/kg) and intramuscular tramadol (Laboratório Teuto Brasileiro, Brazil; 4 mg/mL) and anesthesia was maintained through intubation with 2% isoflurane (Instituto Bioquímico Indústria Farmacêutica, Brazil). The surgery area was trichotomized and embrocated with idiophone solution and isolated with surgical fields. A midline incision was performed on the skin and Sc-AT was extracted from the edges of the surgical wound. After removal of the ovaries, the Vs-AT was extracted from the periovarian region.
Approximately 2 to 4 g of AT were cut into small pieces and washed in phosphate buffered saline (PBS) twice. Then, the pieces were submitted to enzymatic digestion for 40 min at 37℃, in a solution of Dulbecco's modified Eagle's medium (DMEM) low glucose (Gibco, USA) and 0.1 mg/mL of type I collagenase (Gibco, USA). The digested material was centrifuged at 700 × g for 10 min, and the pellet was resuspended in growing media consisting of DMEM, 10% fetal bovine serum (FBS; Gibco, Brazil), and 1% antibiotic (penicillin 1 Unit/mL, streptomycin 1 µg/mL, and L-glutamine 2.92 µg/mL; Gibco, USA) and then seeded in tissue culture flasks (Greiner, Germany). The cultures were incubated at 37℃ with 5% CO2, and every 48 h, the medium was changed. When 80% to 90% confluence was attained, the cultures were treated with trypsin-ethylenediaminetetraacetic acid (EDTA) 0.005% (Gibco, USA) and subcultured.
The Sc- and Vs-AD-MSC phenotypes were evaluated, at passages P4, P6 and P8, by performing flow cytometry as previously described by Yaneselli et al. [34]. Briefly, cells were washed with PBS (Ca++ and Mg++ free) containing 0.5 mM EDTA and 0.5% FBS (FACS-EDTA). Then, cells were immunostained for 30 min at 4℃ in the dark with 10 µL of each of allophycocyanin-conjugated anti-CD44 (clone IM7; Leinco Technologies, USA) and fluorescein isothiocyanate-conjugated anti-CD45/LCA (CD45RO, clone UCHL1; Acris Antibodies, USA) antibodies, as well as 20 µL of phycoerythrin-conjugated anti-CD90 antibody (THY1, clone 5E10; antibodies-online, USA). After incubation, cells were washed with FACS buffer. Acquisition was performed by using a FACS Canto II system (BD Biosciences, USA) with 5,000 events per sample. The obtained data were analyzed by using FlowJo software (Tree Star, USA) and categorized according to the method of Takemitsu et al. [27].
The AD-MSCs at P4, P6, and P8 were seeded in duplicate at 9.4 × 103 cells/well in CELLSTAR 24-well plates (Greiner), and 24 to 48 h later, they were in vitro differentiated with growing media, DMEM low glucose supplemented with 10% FSB and without antibiotic, according to the method of Vieira et al. [30]. Cells were maintained for 21 days with a media change every 3 to 4 days. Briefly, for adipogenic differentiation: 5 µM isobutylmethylxanthine (Sigma-Aldrich, USA), 60 µM indomethacin (Sigma-Aldrich), 1 µM dexamethasone (Sigma-Aldrich), and 5 µg/mL insulin (Eli Lilly and Company, USA) were added to the growing media. Cells were fixed with 4% paraformaldehyde (Sigma-Aldrich) for 15 min and Oil Red O (Sigma-Aldrich) was used to stain the lipid drops. For chondrogenic differentiation: 50 µM ascorbic acid (Sigma-Aldrich), 10 ng/mL transforming growth factor-β (Sigma-Aldrich), and 6.25 µg/mL insulin were added to the growing media. Then, cells were fixed and stained with Alcian Blue (Sigma-Aldrich) to detect the presence of cartilage matrix. For osteogenic differentiation: 1 µM dexamethasone, 10 mM β-glycerophosphate (Sigma-Aldrich), and 50 µM ascorbic acid were added to the growing media. Then, they were fixed and stained with Alizarin Red (Sigma-Aldrich) to detect mineralized matrix.
Nine random microscopic images (640 × 480 pixels, Nikon TE2000-U; Nikon, Japan) were taken at passages P4, P6, and P8 of Alizarin Red-stained Sc- and Vs-origin tissue cultures that had been induced to bone differentiation. Images were analyzed with ImageJ software (National Institutes of Health, USA), which designated pixel status according to the intensity of the red color of the mineralized matrix.
The generalized estimating equation within the IBM SPSS Statistics software (ver. 23.0; IBM, USA) was used to evaluate the potentials of adipogenic, chondrogenic, and osteogenic differentiation and the mineralized matrix synthesis capacity. Results are expressed as mean ± SD values. Differences were considered significant when p < 0.05, and values between p > 0.05 and p < 0.10 were considered as indicative of a tendency.
Cells from both sites had fibroblastic-like morphology, adhered to plastic, and formed homogenous monolayers. AD-MSCs attained 80% confluence at 5 to 7 days. Immunophenotyping of AD-MSCs was assessed at P4, P6, and P8, with similar antigenic surface expression observed among the average passages from the same origin. Both Sc- and Vs-AD-MSCs were positive for CD44 (98.9% ± 0.6% and 97.4% ± 2.3%, respectively). Regarding CD90 expression, 72.3% ± 17.3% of Sc and 71.4% ± 15.8% of Vs cells were positive for this marker. Only 6.0% ± 4.5% of Sc and 4.8% ± 2.7% of Vs cells were positive for CD45, indicating that most cells of both lineages were negative for this hematopoietic marker. In summary, most of the AD-MSCs obtained, regardless of their origin, were positive for CD44 and CD90, with few cells expressing CD45 (Fig. 1).
AD-MSCs of both origins were able to differentiate in vitro into adipose and cartilage lineages (panels A and B in Fig. 2 and panels G and H in Fig. 2). On adipose induction, morphological changes were observed in the first week. At week 3, differentiation was confirmed by the presence of red staining (Oil Red O-positive) of the intracellular lipid drops. At 21 days, the blue color (Alcian Blue-positive) of the chondrogenic-induced cells is due to the presence of glycosaminoglycans. Negative control cells showed no morphological changes and no affinity for dyes, in accordance with their undifferentiated state. Adipogenic and chondrogenic potentials of both lineages (Sc and Vs) diminished with the increase in passages (panels A and B in Fig. 3). Also, Sc cells on passages P4, P6, and P8 showed higher potential for in vitro differentiation than Vs cells; however, these differences were only tendencies (adipose p = 0.091, cartilage p = 0.095). Furthermore, Sc cells showed multipotentiality up to P8, but with a gradual loss of this characteristic and a marked decrease at P6. The majority of the Sc cells grew very little or stopped growing at P8 (category “non-growth”). On the other hand, the Vs-origin cells had plasticity differentiation only up to P4.
The osteogenic-induced cells, at one week after induction, exhibited morphological changes, and presence of a mineralized matrix was confirmed by red staining (Alizarin Red S-positive) (panels C and I in Fig. 2). Sc cells at passages P4, P6, and P8 showed a higher proportion of in vitro differentiation into this lineage than that shown by the Vs-derived cells, but the differences were not significant, only showing a tendency (bone p = 0.091). When this osteogenic differentiation potential was analyzed for both cell origins (Sc and Vs together), there was a negative effect with an increase in passages, but the effect was insignificant (panel C in Fig. 3). The amount of mineralized matrix quantification was compared at P4 of cells from both origins, and the Sc-AD-MSCs produced more mineralized matrix than Vs-AD-MSCs (10.67% ± 2.76% vs. 7.41% ± 2.18%, p < 0.05) (Fig. 4). In addition, between P4 and P8, cells of the Sc lineage showed a significant progressive decrease in mineralization matrix (10.67% ± 2.76% vs. 7.44 ± 1.96% vs. 2.42 ± 2.73%, p < 0.001) (Fig. 5). It was not possible to evaluate the Vs mineralization matrix potential, because of the marked loss of osteogenic potential in sequential passages of Vs cells.
In this study, extraction of AT was performed in a group of young canines undergoing ovariectomy, which is a routine surgery and is usually done on healthy and young animals. It is relatively easy to obtain great amounts of AT for AD-MSCs during that surgery without significant risk for the tissue donor, thus making it such canines a suitable donor population to build a bank of cryopreserved MSCs, which supports comments by other researchers [1617]. In compliance with international recommendations [5], we used the name MSC for cells of both origins that exhibited fibroblastic morphology, plastic-adherence, MSC marker positivity, and in vitro tri-differentiation (adipocytes, chondrocytes, and osteoblasts) ability. AD-MSCs of both origins, Sc and Vs, were positive for CD44 and CD90 and negative for CD45. Other authors have described similar phenotypes in canine AD-MSCs [16242730]. Under our study conditions, more than 90% of the AD-MSCs of Sc and Vs origin expressed CD44, which is similar to results presented by other authors [1527]; although Martinello et al. [16] described a lower CD44 expression level (88% for Vs-AD-MSCs). In contrast, we showed that more than 70% of both AD-MSCs lineages expressed CD90, but other authors have described higher (87% [30]) or lower (35% [16] and 22.55% [27]) expressions. Screven et al. [24] reported higher individual variations and described two categories: one between 10% to 40% expression and other more than 40%. The differences in these studies may be due to the fact that antibodies are not specific for canine markers as they have been designed for mice or humans antigens [222427]. Another reason could be that the cells were evaluated at different passages and were from different origins: Takemitsu et al. [27] worked with AD-MSCs of inguinal Sc origin on P2, Martinello et al. [16] with AD-MSCs of periovaric Vs at passages P3–P6, and Screven et al. [24] on Sc AD-MSCs at P3–P5. These variations in the expression of CD90 have been described in canines [2427] and other species [8121823]. This heterogeneity of expression indicates that anti-CD90 antibodies, designed for humans and mice, may not be appropriate for immunophenotyping canine MSCs. Nevertheless, we observed a high and conserved expression of CD44 at different passages, which supports the results reported by other authors [112730]. We observed a very low expression of CD45, as has also been reported by other authors [162730], which is foreseeable as it is a hematopoietic cell marker.
Some authors have proposed using real-time polymerase chain reaction (PCR) to measure CD44 and CD90 mRNA as an method to avoid the problem of the limited availability of canine-specific MSCs antibodies [1522]. By using flow cytometry, we observed that CD44 and CD90 are expressed at the surface up to P8, similar to the expressions in humans of up to P10 reported by Baglioni et al. [4]. Nevertheless, contradictory results have been reported when using real-time PCR, as CD90 expression decreases at P4 [22], while CD44 and CD90 decrease at P7 [15].
In our work, Sc-origin cells showed a tendency for a higher proportion of differentiation than Vs-origin cells (adipose p = 0.091, cartilage p = 0.095, and bone p = 0.091). Other authors describe similar multipotentialities between those two origins [49]. The tendencies in our results might become differences if the study is repeated with more animals. In our study, adipose differentiation of canine Sc- and Vs-AD-MSCs, revealed few and small lipid vacuoles, similar as the results described by Vieira et al. [30]. Even though other authors do not express concern about these vacuole characteristics in the text, the pictures in their publications reveal very few lipid vacuoles [1516]. On the other hand, it might not be a difference related to the species being studied, as Guercio et al. [9] have described canine cells having big and several lipid vacuoles, as reported in humans [4] and mice [28]. The different results observed may also be due to the formulations of the induction media used. Moreover, it has been suggested that classical histochemical staining for detecting adipose differentiation should be considered in the measurement of genic expression biomarkers [515].
Regarding the quantification of in vitro mineralized matrix, significant differences were observed, with the Sc-AD-MSCs showing a higher mineralized matrix percentage than the Vs-AD-MSCs (10.67% ± 2.76% vs. 7.41% ± 2.18%). Other researchers have reported similar results, Sc-derived cells had more gene expression of biomarkers (COLIA1 and RUNX2) of bone differentiation in canine [22] and humans AD-MSCs [3]. However, Baglioni et al. [4] did not find different capacities of mineralized matrix synthesis in human AD-MSCs from Sc and Vs sites at passages P1–P4. Results contrasting ours were obtained in rabbits by Peptan et al. [21] who reported a higher proportion of mineralized matrix in Vs than in Sc cells (35.25% ± 6.36% vs. 15.16% ± 3.51%). These study differences could be due to the use of different species and/or passages as Peptan et al. [21] analyzed P1 (not P4) and the stain used was von Kossa (not Alizarin Red S). However, Kang et al. [11] reported differences in in vitro osteogenic potential but did not detect it in vivo, suggesting that to measure this potential in canines it is more appropriate to use an in vivo model.
Our results show that MSCs of Sc and Vs origins behave differently in regard to in vitro plasticity. The Vs lineage exhibited tri-differentiation up to P4, while tri-differentiation in Sc cells was present up to P8, with an accentuated reduction between P6 and P8. A loss of multilineage differentiation potential in subculturing has been observed for canine AD-MSCs by others [915]. In our results, the predominant category at P8 was “non-growth”, which could be due to the cells entering senescence. Martinello et al. [16] described a marked decrease in telomerase activity in canine AD-MSCs from P8 onward, indicating that cells started undergoing senescence. Requicha et al. [22] reported that, by assessing biomarker gene expression, canine AD-MSCs start losing multipotentiality at P3. Other authors have reported that canine cells conserve plasticity up to P6 [915], which is in agreement with our results showing that the Sc lineage underwent a large decrease between P6 and P8. Nevertheless, Zuk et al. [35] showed that human cells start undergoing senescence at P15, showing that there are differences between species in the longevity of cultures. We believe that it is clinically important and relevant for regenerative therapeutics in animal model or veterinary medicine, to determine at what passage the MSCs conserve their stemness capacity. Such beliefs have been expressed by others [915].
We observed a difference in plasticity of AD-MSCs based on AT origin, with Sc-derived MSCs differentiating at higher passages than Vs MSCs. The hypothesis that cells have memory of the site of extraction, might explain the differences detected in our study, differences that support observations reported by others [3922]. The basis of this hypothesis derives from the metabolism of glucose in a diabetes murine model, in which Sc-AT is more efficient than Vs-AT in glucose metabolism [1029].
In conclusion, our results show that the Sc-AD-MSC lineage has advantages over the Vs-AD-MSC lineage due to Sc-AD-MSCs having tri-differentiation capacity at higher passages, a higher in vitro bone mineralized matrix synthesis capacity, and a less invasive method of AT extraction. Although working with Sc-AD-MSCs in culture is more practical and efficient than working with Vs-AD-MSCs, more research is necessary to determine whether the differences observed in vitro are maintained in vivo.
Figures and Tables
Fig. 1
Expressions of CD44, CD90, and CD45 on adipose tissue-derived mesenchymal stem cells of subcutaneous (Sc) or visceral (Vs) origin were assessed by performing flow cytometry (n = 2). Data are representative of analyzed passages.
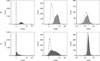
Fig. 2
Tri-differentiation microscopic images of adipose tissue-derived mesenchymal stem cells (AD-MSCs) at P2 in vitro (n = 5). Adipogenic differentiation images at 400× (intracellular lipid vacuoles) for subcutaneous (Sc) and visceral (Vs) (A and G). Chondrogenic differentiation images at 40× (B and H). Osteogenic differentiation images at 40× (C and I). Negative controls are shown in images D–F and J–L.
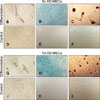
Fig. 3
Evaluation of in vitro differentiation of adipose tissue-derived mesenchymal stem cells from both extraction sites at different passages (n = 5). (A) Adipogenic differentiation. (B) Chondrogenic differentiation. (C) Osteogenic differentiation. The “non-growth” category exhibits an initial gradual increase, becoming abrupt between P6 to P8. The multipotentiality had a negative effect on adipose (p < 0.001) and cartilage (p < 0.01) lineages, but the bone lineage showed a marked tendency with the increase of passages (p = 0.054).
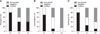
Fig. 4
In vitro bone matrix synthesis capacity of adipose tissue-derived mesenchymal stem cells of subcutaneous (Sc) and visceral (Vs) origins at P4 (n = 5). Macroscopic and microscopic (40×) images of Sc (A and B) and Vs (C and D) cells. The Sc cells showed a greater capacity for matrix synthesis than that shown by Vs cells (E). *p < 0.05.
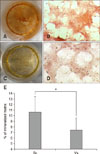
Acknowledgments
This research was partially supported by the Agencia Nacional de Investigación e Innovación (ANII), Uruguay and the Coordenação de Aperfeiçoamento de Pessoal de Nivel Superior and Universidad de la República (CAPES-UdelaR) and Centro de Pesquisa Experimental, Hospital de Clínicas de Porto Alegre (CPE-HCPA), Brazil.
References
1. An SY, Han J, Lim HJ, Park SY, Kim JH, Do BR, Kim JH. Valproic acid promotes differentiation of hepatocyte-like cells from whole human umbilical cord-derived mesenchymal stem cells. Tissue Cell. 2014; 46:127–135.


2. Anderson P, Souza-Moreira L, Morell M, Caro M, O'Valle F, Gonzalez-Rey E, Delgado M. Adipose-derived mesenchymal stromal cells induce immunomodulatory macrophages which protect from experimental colitis and sepsis. Gut. 2013; 62:1131–1141.


3. Baglioni S, Cantini G, Poli G, Francalanci M, Squecco R, Di Franco A, Borgogni E, Frontera S, Nesi G, Liotta F, Lucchese M, Perigli G, Francini F, Forti G, Serio M, Luconi M. Functional differences in visceral and subcutaneous fat pads originate from differences in the adipose stem cell. PLoS One. 2012; 7:e36569.


4. Baglioni S, Francalanci M, Squecco R, Lombardi A, Cantini G, Angeli R, Gelmini S, Guasti D, Benvenuti S, Annunziato F, Bani D, Liotta F, Francini F, Perigli G, Serio M, Luconi M. Characterization of human adult stem-cell populations isolated from visceral and subcutaneous adipose tissue. FASEB J. 2009; 23:3494–3505.


5. Bourin P, Bunnell BA, Casteilla L, Dominici M, Katz AJ, March KL, Redl H, Rubin JP, Yoshimura K, Gimble JM. Stromal cells from the adipose tissue-derived stromal vascular fraction and culture expanded adipose tissuederived stromal/stem cells: a joint statement of the International Federation for Adipose Therapeutics and Science (IFATS) and the International Society for Cellular Therapy (ISCT). Cytotherapy. 2013; 15:641–648.


7. Chen J, Li Y, Katakowski M, Chen X, Wang L, Lu D, Lu M, Gautam SC, Chopp M. Intravenous bone marrow stromal cell therapy reduces apoptosis and promotes endogenous cell proliferation after stroke in female rat. J Neurosci Res. 2003; 73:778–786.


8. Gronthos S, Franklin DM, Leddy HA, Robey PG, Storms RW, Gimble JM. Surface protein characterization of human adipose tissue-derived stromal cells. J Cell Physiol. 2001; 189:54–63.


9. Guercio A, Di Bella S, Casella S, Di Marco P, Russo C, Piccione G. Canine mesenchymal stem cells (MSCs): characterization in relation to donor age and adipose tissue-harvesting site. Cell Biol Int. 2013; 37:789–798.


10. Hocking SL, Chisholm DJ, James DE. Studies of regional adipose transplantation reveal a unique and beneficial interaction between subcutaneous adipose tissue and the intra-abdominal compartment. Diabetologia. 2008; 51:900–902.


11. Kang BJ, Ryu HH, Park SS, Koyama Y, Kikuchi M, Woo HM, Kim WH, Kweon OK. Comparing the osteogenic potential of canine mesenchymal stem cells derived from adipose tissues, bone marrow, umbilical cord blood, and Wharton's jelly for treating bone defects. J Vet Sci. 2012; 13:299–310.


12. Katz AJ, Tholpady A, Tholpady SS, Shang H, Ogle RC. Cell surface and transcriptional characterization of human adipose-derived adherent stromal (hADAS) cells. Stem Cells. 2005; 23:412–423.


13. Krasnodembskaya A, Song Y, Fang X, Gupta N, Serikov V, Lee JW, Matthay MA. Antibacterial effect of human mesenchymal stem cells is mediated in part from secretion of the antimicrobial peptide LL-37. Stem Cells. 2010; 28:2229–2238.


14. Laschke MW, Harder Y, Amon M, Martin I, Farhadi J, Ring A, Torio-Padron N, Schramm R, Rücker M, Junker D, Häufel JM, Carvalho C, Heberer M, Germann G, Vollmar B, Menger MD. Angiogenesis in tissue engineering: breathing life into constructed tissue substitutes. Tissue Eng. 2006; 12:2093–2104.


15. Lee KS, Kang HW, Lee HT, Kim HJ, Kim CL, Song JY, Lee KW, Cha SH. Sequential sub-passage decreases the differentiation potential of canine adipose-derived mesenchymal stem cells. Res Vet Sci. 2014; 96:267–275.


16. Martinello T, Bronzini I, Maccatrozzo L, Mollo A, Sampaolesi M, Mascarello F, Decaminada M, Patruno M. Canine adipose-derived-mesenchymal stem cells do not lose stem features after a long-term cryopreservation. Res Vet Sci. 2011; 91:18–24.


17. Mitchell A, Rivas KA, Smith R 3rd, Watts AE. Cryopreservation of equine mesenchymal stem cells in 95% autologous serum and 5% DMSO does not alter post-thaw growth or morphology in vitro compared to fetal bovine serum or allogeneic serum at 20 or 95% and DMSO at 10 or 5%. Stem Cell Res Ther. 2015; 6:231.


18. Mitchell JB, McIntosh K, Zvonic S, Garrett S, Floyd ZE, Kloster A, Di Halvorsen Y, Storms RW, Goh B, Kilroy G, Wu X, Gimble JM. Immunophenotype of human adipose-derived cells: temporal changes in stromal-associated and stem cell-associated markers. Stem Cells. 2006; 24:376–385.


19. Niemeyer P, Szalay K, Luginbühl R, Südkamp NP, Kasten P. Transplantation of human mesenchymal stem cells in a non-autogenous setting for bone regeneration in a rabbit critical-size defect model. Acta Biomater. 2010; 6:900–908.


20. Nijnik A, Hancock RE. The roles of cathelicidin LL-37 in immune defences and novel clinical applications. Curr Opin Hematol. 2009; 16:41–47.


21. Peptan IA, Hong L, Mao JJ. Comparison of osteogenic potentials of visceral and subcutaneous adipose-derived cells of rabbits. Plast Reconstr Surg. 2006; 117:1462–1470.


22. Requicha JF, Viegas CA, Albuquerque CM, Azevedo JM, Reis RL, Gomes ME. Effect of anatomical origin and cell passage number on the stemness and osteogenic differentiation potential of canine adipose-derived stem cells. Stem Cell Rev. 2012; 8:1211–1222.


23. Riekstina U, Cakstina I, Parfejevs V, Hoogduijn M, Jankovskis G, Muiznieks I, Muceniece R, Ancans J. Embryonic stem cell marker expression pattern in human mesenchymal stem cells derived from bone marrow, adipose tissue, heart and dermis. Stem Cell Rev. 2009; 5:378–386.


24. Screven R, Kenyon E, Myers MJ, Yancy HF, Skasko M, Boxer L, Bigley EC 3rd, Borjesson DL, Zhu M. Immunophenotype and gene expression profile of mesenchymal stem cells derived from canine adipose tissue and bone marrow. Vet Immunol Immunopathol. 2014; 161:21–31.


25. Strem BM, Hicok KC, Zhu M, Wulur I, Alfonso Z, Schreiber RE, Fraser JK, Hedrick MH. Multipotential differentiation of adipose tissue-derived stem cells. Keio J Med. 2005; 54:132–141.


26. Szaraz P, Librach M, Maghen L, Iqbal F, Barretto TA, Kenigsberg S, Gauthier-Fisher A, Librach CL. In vitro differentiation of first trimester human umbilical cord perivascular cells into contracting cardiomyocyte-like cells. Stem Cells Int. 2016; 2016:7513252.
27. Takemitsu H, Zhao D, Yamamoto I, Harada Y, Michishita M, Arai T. Comparison of bone marrow and adipose tissue-derived canine mesenchymal stem cells. BMC Vet Res. 2012; 8:150.


28. Terraciano P, Garcez T, Ayres L, Durli I, Baggio M, Kuhl CP, Laurino C, Passos E, Paz AH, Cirne-Lima E. Cell therapy for chemically induced ovarian failure in mice. Stem Cells Int. 2014; 2014:720753.


29. Tran TT, Yamamoto Y, Gesta S, Kahn CR. Beneficial effects of subcutaneous fat transplantation on metabolism. Cell Metab. 2008; 7:410–420.


30. Vieira NM, Brandalise V, Zucconi E, Secco M, Strauss BE, Zatz M. Isolation, characterization, and differentiation potential of canine adipose-derived stem cells. Cell Transplant. 2010; 19:279–289.


31. Volk SW, Theoret C. Translating stem cell therapies: the role of companion animals in regenerative medicine. Wound Repair Regen. 2013; 21:382–394.


33. Wu Y, Chen L, Scott PG, Tredget EE. Mesenchymal stem cells enhance wound healing through differentiation and angiogenesis. Stem Cells. 2007; 25:2648–2659.


34. Yaneselli K, Filomeno A, Semiglia G, Arce C, Rial A, Muñoz N, Moreno M, Erickson K, Maisonnave J. Allogeneic stem cell transplantation for bone regeneration of a nonunion defect in a canine. Vet Med Res Rep. 2013; 4:39–44.