Abstract
In Korea, several outbreaks of low pathogenic AI (H9N2) viral infections leading to decreased egg production and increased mortality have been reported on commercial farms since 1996, resulting in severe economic losses. To control the H9N2 LPAI endemic, the Korea Veterinary Authority has permitted the use of the inactivated H9N2 LPAI vaccine since 2007. In this study, we developed a killed vaccine using a low pathogenic H9N2 AI virus (A/chicken/Korea/ADL0401) and conducted safety and efficacy tests in commercial layer farms while focusing on analysis of factors that cause losses to farms, including egg production rate, egg abnormality, and feed efficiency. The egg production rate of the control group declined dramatically 5 days after the challenge. There were no changes in feed consumption of all three groups before the challenge, but rates of the control declined afterward. Clinical signs in the vaccinated groups were similar, and a slight decline in feed consumption was observed after challenge; however, this returned to normal more rapidly than the control group and commercial layers. Overall, the results of this study indicate that the safety and efficacy of the vaccine are adequate to provide protection against the AI field infection (H9N2) epidemic in Korea.
The low pathogenic avian influenza (LPAI) H9N2 virus is prevalent in poultry worldwide, and its antibody was detected in humans in Hong Kong in 1997 [6]. In the poultry industry, the LPAI H9N2 viral serotype is prevalent in Asian countries, including Korea, Hong Kong, China, Japan, Pakistan, Iran, Vietnam, Indonesia, and Cambodia. The main symptoms associated with this viral infection include egg drop syndrome, respiratory problems and occasional mortality in poultry, including chicken and turkey; accordingly this virus causes large economic losses to the poultry industry [21424]. Studies of vaccines against high pathogenic avian influenza (HPAI) virus and LPAI are currently being performed in many countries. Vaccines are broadly classified into live and inactivated vaccines; however, live vaccines are rarely used in many countries owing to the potential for viral contamination and genetic mutations. Most inactivated vaccines have been developed based on the viral strain prevalent in each occurrence area.
To develop an oil emulsion vaccine (OE), a vaccine virus is inoculated into chicken embryos, which are then inactivated and emulsified. The results of laboratory and field assessments of OE vaccines have shown that they do not cause infections, but that they prevented clinical symptoms and the associated decrease in egg production in response to challenge by the same serotype of the avian influenza (AI) virus 2 to 3 weeks after vaccination [26].
Since 16 different HA serotypes that exhibit little or no cross-protection exist, the HA serotype identical to the field virus needs to be included in the vaccine for efficacy. Neuraminidase (NA), which is another viral surface antigen, has shown immune-competence during challenges by field viruses with the same NA but different HA types [19]. Therefore, the HA serotype of the vaccine and field challenge virus should be identical to provide sufficient protection against infection in the field. However, inoculation with an OE vaccine has the drawback of generating positive results during the hemagglutination inhibition (HI) test and agar gel immunoprecipitation (AGP). Therefore, serological discrimination must be achieved by employing NA inhibition when the viral NA used in the vaccine and that used during the challenge inoculation differ.
The inactivated AI pox-vectored vaccine was developed in the past by inserting a HA gene of the AI virus into the pox virus [31027]. Inoculation of this vaccine into chickens generated antibodies against the pox virus along with HA AI viral proteins. Therefore, inoculation of the pox vaccine prior to AI pox-vectored vaccine inoculation is limited by decreased efficacy due to in vivo inhibition [27]. However, chickens inoculated with the AI pox-vectored vaccine tested negative during the AGP tests, which are used to detect nucleoprotein or matrix 1 proteins of the AI virus. This is advantageous when discriminating between a field infection and a vaccine reaction. In addition, the HI antibody titer against AI in chickens administered the AI pox-vectored vaccine was very low; however, this titer is clinically sufficient to protect against an HPAI viral challenge and has been successfully used in Mexico [3].
In Korea, several outbreaks of the LPAI H9N2 infection have been reported by domestic commercial poultry farms, causing decreased egg production, increased mortality, and severe economic losses since 1996 [15161721]. To control endemic H9N2 LPAI infections, the Korean Veterinary Authority permitted the use of the oil-based inactivated H9N2 LPAI vaccines in 2007 [7]. Therefore, in this study we evaluated a killed vaccine using the LPAI H9N2 virus (ADL0401) isolated in Korea and conducted safety and efficacy evaluations in commercial layers. This study focused on analysis of factors that cause severe losses to farms, such as seroresistance, low egg production, abnormal egg detection, and low feed intake, as well as abnormal eggshell and egg yolk color, which are closely related to the LPAI clinical symptoms.
The vaccine candidate strain used was the A/Chicken/ Korea/ADL0401 (H9N2) field strain isolated from the excrement and cloaca originating from poultry layer farms in Korea suspected of being LPAI contaminated. The Veterinary Department of Chungbuk University isolated the viral strain, which was used as the master seed at the Choongang Vaccine Laboratory in Daejeon, Korea, where the vaccine was further developed. The H9N2 vaccine candidate virus (108.3 egg infective dose50 [EID50]/mL) was propagated in 10-day-old SPF eggs at 37℃ for 72 h. Allantoic fluid was harvested, and the vaccine strain was inactivated at 4℃ for 24 h using 0.1% formalin. The fluid containing the inactivated virus was conjugated with the oil adjuvant (Montanide ISA 70; SEPPIC, France) in a 3 : 7 ratio. The A/Chicken/Korea/MS96 (H9N2) used as the challenge virus was obtained from the Animal and Plant Quarantine Agency (QIA) in Anyang, Korea.
Oral and cloacal swab samples were collected from layer hens following the test and placed in 15 mL conical tubes containing streptomycin (2 mg/mL), penicillin (2,000 IU), and kanamycin (0.25 mg) in phosphate-buffered saline (PBS), after which they were thoroughly mixed. The tissue samples analyzed were first weighed, then ground in a sterilized mortar with a small amount of silica sand. The ground samples were also added to a mixture of the same antibiotics in PBS. Next, the pre-treated samples and tissues were centrifuged at 2,100 × g for 10 min, after which the supernatants were collected and inoculated into the allantoic sac of 1-day-old SPF eggs at a dose of 0.2 mL [12].
Twenty-five microliters of serum were placed in a 96-well microtiter plate. After two-fold dilutions in PBS, 25 µL of the H9 type-specific antigen was added and mixed thoroughly. After incubating for 20 min at room temperature, 25 µL of a 1% suspension of chicken erythrocytes was added, mixed thoroughly, and the hemagglutination-inhibition (HI) titers were determined following 40-min of incubation at room temperature [1]. The H9 type-specific antigen and positive serum were obtained from the QIA, and four HA units were used in each well of the culture plate for the HI test.
Quantitative reverse transcriptase-polymerase chain reaction (qRT-PCR) analysis was used to determine the LPA1 viral gene expression levels. The correlations between the viral concentration and measurements were determined as follows. The gene of the LPAI virus was extracted at a titer of 107.9 embryo infective dose 50% (EID50)/mL and diluted following the decimal scale. Each diluted sample was quantified using the qRT-PCR method, after which the threshold cycle value was calculated. The viral RNA was extracted using the viral Gene-Spin Viral DNA/RNA extraction kit (iNtRON Biotechnology, Korea) according to the manufacturer's protocol. qRT-PCR analysis of the extracted viral RNA was conducted using the I-AIV (H9) detection kit (iNtRON Biotechnology). Briefly, 2 µL of the RNA template and 8 µL of the enzyme mix were added to the diagnosis solution to achieve a total reaction volume of 20 µL. The PCR procedure was as follows: RT at 45℃ for 30 min, initial denaturation at 94℃ for 5 min, followed by 50 cycles of denaturation at 94℃ for 30 sec, annealing at 50℃ for 30 sec, extension at 72℃ for 40 sec, and then final extension at 72℃ for 5 min. The reaction product was amplified using a Thermo Hybrid PCR express thermal cycler (UK), after which the amplification product was separated using 1.2% agarose gel electrophoresis (Invitrogen, USA). The size of the amplified H9 gene was confirmed using the standard 1 kb Plus DNA Ladder (Invitrogen). The specific band of interest was observed by visualization with a 5× loading dye.
For the vaccine safety tests, 50 of the 47-week-old laying hens from the once- (100-day-old, egg drop treatment1 [EDTA1]) and twice-vaccinated groups (45- and 100-day-old, EDT2), as well as 50 of the 47-week-old laying hens from the control group that tested negative in the serological AI analysis were selected. The chickens were evaluated after they were transferred to an experimental farm that was designed for this test to enable disinfection. Birds were observed for clinical symptoms daily, and tissues were collected from the inoculated area as well as blood samples after completion of the test to observe the reactions in these tissues.
For the vaccine efficacy tests, the eggs were collected during a specific period after the challenge. Water was provided ad libitum, while a consistent amount of feed was provided daily, and the residual feed was weighed daily. Blood samples were also collected following the designated schedules and the egg production, abnormal egg detection, and feed intake, as well as changes in the eggshell and egg yolk color were investigated and recorded periodically. The efficacy of the developed vaccine was evaluated using the serological examinations described under the serum test in the Materials and Methods section. The HI titers of the sera from the EDT1 and EDT2 flocks on the target commercial farms were determined and compared with those of the control flocks.
All data were analyzed using an independent t-test to identify differences between groups using the statistical package for the social sciences (SPSS) program for Windows (ver. 20; IBM, USA). In addition, general linear model (GLM) analysis incorporating the one-, two-, and three-way analysis of variance (ANOVA) models was performed. A p < 0.05 indicated statistical significance.
The test flocks were monitored twice a day for clinical signs and symptoms during the test period. Mild respiratory symptoms and diarrhea were observed in 20 and 13% of the EDT1 and EDT2 flocks, respectively, after challenge. There was no significant difference between flocks (p > 0.05). However, the EDT1 and EDT2 flocks recovered sooner than the non-vaccinated control flocks, which exhibited severe respiratory symptoms and diarrhea on day 3 following the challenge and failed to recover until the end of the test (Table 1).
The HI titers of the EDT1, EDT2, and non-vaccinated control flocks were compared before and after the challenge (Table 2, Fig. 1). The titers of the EDT1 and EDT2 flocks prior to challenge were 7.0 ± 1.0 (log2) and 7.6 ± 0.7 (log2), respectively, which are sufficiently protective levels. The titer of the EDT2 flock was higher than that of EDT1, while no antibodies were detected in the non-vaccinated control group.
The changes in HI titer were not evident in the EDT1 flocks by day 5 after the challenge, but had elevated to 7.9 ± 0.3 (log2) by day 14. In addition, the HI titers of the EDT1 flocks were significantly different prior to and after challenge (p < 0.05). The HI titer of the EDT2 flocks was already high prior to challenge and was around 8.0 ± 0.0 (log2) on day 5 and 14 after challenge, which was not a large increase. However, these titer values were significantly different prior to and after the challenge. Furthermore, the HI titer of the non-vaccinated control flocks increased to 6.3 ± 1.2 (log2), which confirmed that the challenge virus was adequately administered.
In this test, birds from the EDT1 and EDT2 flocks were randomly selected and challenged with the virus. The levels of cloacal virus shedding were chemically analyzed using qRT-PCR. The presence of AIV was detected on day 3 after challenge in samples from EDT1 and EDT2, as well as the non-vaccinated control flocks, confirming the presence of infections (Table 3). Viral concentrations from the mouth and cloaca of the EDT1 flocks were 2.4 ± 2.8 and 1.6 ± 2.1 EID50, respectively. On day 5 after vaccination, the oral viral concentration detected was 2.3 ± 2.6 EID50, but no virus was detected in the kidney or cloaca. However, the viral concentration of the EDT1 flock was much lower than that of the non-vaccinated control flock.
Viral concentrations of 1.3 ± 1.7 EID50 were detected in the EDT2 flock on day 5 after vaccination, but this was considered an extremely low value. An oral viral gene concentration of 4.2 ± 4.1 EID50 was detected in the non-vaccinated control flocks on day 3 after challenge, which was very high. However, this value consistently declined from day 5 after challenge, and was undetectable by day 10. No viral genes were detected in the cloaca on day 3 after challenge, but levels were extremely high by day 5 (3.1 ± 3.3 EID50). The EDT2 flocks clearly shed fewer viruses than EDT1, and the difference between the vaccinated and non-vaccinated flocks was extremely high (Table 3).
The egg production of the EDT1, EDT2, and non-vaccinated control flocks were compared in this test (Fig. 2). The EDT1 flock, which showed an egg production rate of 86% prior to challenge, experienced a 10% decline on day 7 after the challenge, but the levels had returned to normal by the next day. Therefore, we determined that the change in egg production was not associated with the effects of the challenge virus. The egg production rate of the EDT2 flock was 70% before the challenge and increased to 72 to 86% afterward (p < 0.05), while that of the non-vaccinated control group was 83% before and declined to 67% by day 4 after the challenge. The non-vaccinated control flock maintained an egg production rate of 67 to 74% up to the end of the test period, and the decline was significant (p < 0.05) compared to the vaccinated groups.
In summary, the changes induced by the challenge in the EDT1 flock were minor, and the egg production recovered rapidly. The egg production in the EDT2 flock increased by about 2 to 11% after challenge, but the decline in production was clear in the non-vaccinated control flock and was about 13 to 16% on day 4 after the challenge.
This test compared the production of abnormal eggs by the EDT1, EDT2, and non-vaccinated control flocks (Fig. 3). Abnormal eggs were determined as low-quality eggs that were nutritionally deficient, decolorized, damaged, and had abnormal eggshells. Normal flocks also produce abnormal eggs, and the rate considered normal in domestic flocks is below 5% [23]. The abnormal egg production rates of the EDT1 and EDT2 flocks were all below 5% prior to and after the challenge. The nonvaccinated control flocks showed an abnormal egg production rate of 3.3%, which was within the normal range, but this increased to 6.7 to 18.5% after challenge (p < 0.05). The results showed there was a clear difference in the production of abnormal eggs by the vaccinated and non-vaccinated control flocks.
The feed intake of the EDT1, EDT2, and non-vaccinated control flocks was compared in this test (Fig. 4). The EDT1 and EDT2 flocks showed a 6 to 10% decline in feed intake from day 4 to 11 after the challenge, but their recovery period was considerably shorter than that of the non-vaccinated control flock. The feed intake of the non-vaccinated control flock started to decline on day 3 after challenge, and was reduced significantly (p < 0.05) by 10 to 34% by day 14. Although there were differences in feed intake between the flocks in different conditions of health, there was a clear difference between the vaccinated (EDT1 and EDT2) and non-vaccinated control flocks.
The eggshell colors of the EDT1, EDT2, and non-vaccinated control flocks were compared using the eggshell color fan (DSM color fan; Orka Technology, USA) in this test (Fig. 5). The EDT1 and EDT2, which were on level 12 of the eggshell color fan before the challenge, dropped to level 11 by day 5 after the challenge. The non-vaccinated control flock was also on level 12 before and had dropped to between levels 8 and 11 by day 5 after the challenge. The eggshell color fan of the EDT1 and EDT2 flocks dropped 1 level by day 5 after the challenge, while the non-vaccinated control flock dropped 2 to 4 levels by day 5 after challenge.
The egg yolk color of the EDT1, EDT2, and non-vaccinated control flocks were compared in this test (Fig. 6). The EDT1 and EDT2 flocks, which were on level 9 of the egg yolk color fan before the challenge, dropped to level 8 by day 3 after the challenge. The non-vaccinated control was on level 8 before and had dropped to level 7 by day 4 after the challenge. The egg yolk color of the EDT1, EDT2, and non-vaccinated control flocks all dropped 1 level after challenge.
In the present study, separate EDT1 and EDT2 flocks of common broilers were inoculated once or twice, respectively, with the LPAI H9N2 killed oil vaccine. These flocks were then challenged with the LPAI MS96 (H9N2) virus and various parameters, including the development of clinical signs, change in antibody titer, virus shedding, change in egg production, production of abnormal eggs and feed intake, as well as changes in the eggshell and yolk color were determined. The qRT-PCR method was used to analyze the virus shedding quantities.
A number of studies have focused on the clinical signs that manifest when LPAI-vaccinated flocks are challenged with a virus or experience field infections [1118]. In Iran, the LPAI H9N2 killed oil vaccine was inoculated subcutaneously into 10-day-old broilers, which were then challenged with the H9N2 virus at 21 days of age. Clinical signs including anorexia, reduced water intake, depression, and tussis were observed in the vaccinated flocks, but there were no deaths [1322]. However, in our study, we did not observe any clinical signs in the vaccinated EDT1 and EDT2 flocks. There are two possible explanations for the apparent discrepancy between these results; specifically, there may be differences in the virulence of the challenge virus or in the efficacy of the locally developed LPAI H9N2 killed oil vaccine used in our study, which could have contributed to alleviating clinical signs. However, the manifestation of clinical signs such as diarrhea and respiratory problems in the non-vaccinated control flock (Table 1) coupled with evidence that the virus shedding was much lower in the vaccinated flocks points more to the efficacy of the vaccine playing a role.
A study conducted in Italy revealed that the HI titers developed to a level of 9.0 (log2) 4 weeks after the LPAI H7N2 killed oil vaccine was administered [20]. In addition, a study in the US showed that flocks administered the LPAI H7N2 killed oil vaccine once (1-day-old) and twice (1- and 4-weeks-old) developed HI titers of 5.0 (log2) and 7.0 (log2), respectively, 4 weeks after vaccination [28]. The HI titers of the EDT1 and EDT2 flock observed in this study before the MS96 (H9N2) challenge showed that the flocks were adequately protected against the H9N2 AI virus. The HI titers of the EDT1 and EDT2 flocks did not increase dramatically following challenge, while that of the non-vaccinated control flock did. Similar to previous reports, the changes in the HI titer were not high in the vaccinated flocks after challenge in this study.
In a study conducted by an affiliated laboratory of the US Department of Agriculture, the H5N1 virus was isolated from the cloaca and mouth of a flock that was inoculated with the LPAI H5N2 killed oil vaccine 2 days after they were subjected to a challenge [27]. However, 6 days after the challenge, no virus was isolated from the cloaca or mouth of the flocks vaccinated with the LPAI vaccine [415], and similar results were obtained in this study. On day 3 after challenge, the EDT1 flock showed evidence of oral and cloacal viral presence, and by day 5 the virus was only detected orally. The EDT2 flock showed only low oral viral detection 5 days after the challenge. These results clearly show that virus shedding was more dramatically reduced in the EDT2 flock that was twice-vaccinated than in the EDT1 flock. Therefore, our results suggest that the administration of the killed vaccines is highly effective at decreasing the clinical signs and virus shedding of flocks (Table 3).
Studies have reported changes in egg production induced by LPAI infections [31]. A study using domestically isolated LPAI MS96 in a challenge administering the virus through the nasal cavity to 86-week-old broilers and 30-week-old layers revealed a 30 to 40% decrease in egg production [15]. A study conducted in another country reported that clinical symptoms such as anorexia, depression, and a 3 to 10% drop in egg production were observed when common broilers were infected with LPAI H9N2 [5]. In the present study, the egg production of the EDT1 flock decreased 3 days after challenge, but was maintained at an acceptable level until the end of the study.
The egg production of the EDT2 flock, which was at an acceptable level of 70%, increased slightly to 73% 3 days after challenge and further increased to a steady and higher rate of around 72 to 86% until the study ended. No virus challenge-related drop in egg production was observed in the vaccinated groups. Conversely, the non-vaccinated control flock, which had a high rate of egg production (85%) prior to the challenge, started to show a decline 4 days after challenge. The egg production further declined to a rate considerably lower than observed before the challenge (67–74%) to the end of the study and failed to recover (Fig. 2). The changes in egg production we observed were similar to previous reports, and it is apparent that these declines can be prevented by vaccination.
Abnormal eggs are determined as low-quality eggs that are nutritionally deficient and decolorized. There is a high possibility of the production of abnormal eggs by flocks infected with infectious bronchitis and egg drop syndrome 1976 (EDS'76) [8925]. In this study, abnormal egg production in the EDT1 and EDT2 flocks was below 5%, which is considered normal by domestic standards [23]. In the non-vaccinated control flock, abnormal egg production prior to challenge was 3.3%, which was in the normal range, while this was significantly elevated to 6.7 to 18.5% after challenge. These changes were similar to the abnormal egg production that often occurs in common broilers infected by field AI. Therefore, we concluded that this study adequately simulated conditions in the field.
Anorexia or reduction in feed intake is a common clinical sign that occurs after AI infections. There were slight signs of anorexia in the vaccinated EDT1 and EDT2 flocks after challenge. However, the non-vaccinated control flock showed a dramatic decrease in feed intake of 10 to 34% that started 3 days after challenge, continued until the end of the study and failed to recover afterward. These changes were similar to those that occur under field conditions and therefore corroborated the results that suggested vaccination provided adequate protection to flocks.
Change in eggshell color is common in infections with Newcastle disease [2930]. The EDT1 and EDT2 flocks exhibited a level 12 eggshell color before challenge, and this dropped by 1 level afterward. The non-vaccinated control flock, which was also at level 12 before challenge, dropped 4 color levels afterwards. Therefore, these results suggest that AI infections also cause changes in eggshell colors.
The changes in egg yolk color were also analyzed, although they are not common in AI infections. The EDT1 and EDT2 flocks, which were at level 9 of the egg yolk color fan before challenge, remained in the range of levels 8 to 9 afterward. The non-vaccinated control flock, which was at level 8 before the challenge, maintained its eggshell color between levels 6 and 8 afterward. These changes were not significantly different.
In conclusion, the clinical signs that commonly occur in field infections were compared to what we observed in the vaccinated and non-vaccinated experimental flocks. There were clear differences in egg production, feed intake, and eggshell color between flocks. In addition, it was apparent that the vaccinated groups with a higher HI titer were more considerably protected against virus shedding than the non-vaccinated group. Therefore, it can be concluded that the administration of the newly developed LPAI H9N2 vaccine to common broilers can provide adequate protection against field infections.
Figures and Tables
Fig. 1
Comparison of hemagglutination inhibition (HI) titer of flocks after MS96 (H9N2) challenge. EDC, non-vaccinated flock.
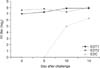
Fig. 5
Comparison of egg-shell color of flocks after MS96 (H9N2) challenge. Eggshell color fan number ranged from 1 to 15.
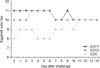
Fig. 6
Comparison of egg yolk color of flocks after MS96 (H9N2) challenge. Egg yolk color fan number ranged from 1 to 15.
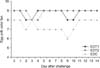
Table 1
Comparison of clinical signs and hemagglutination inhibition (HI) titer of commercial layers after MS96 (H9N2) challenge

Acknowledgments
The authors thank the Animal and Plant Quarantine Agency of Korea for providing the H9N2 AI virus (MS96).
References
1. Allan WH, Lancaster JE, Tóth B. Newcastle Disease Vaccines: Their Production and Use. Rome: Food and Agriculture Organization of the United Nations;1978. p. 54–94.
2. Banks J, Speidel EC, Harris PA, Alexander DJ. Phylogenetic analysis of influenza A viruses of H9 haemagglutinin subtype. Avian Pathol. 2000; 29:353–359.


3. Beard CW, Schnitzlein WM, Tripathy DN. Protection of chickens against highly pathogenic avian influenza virus (H5N2) by recombinant fowlpox viruses. Avian Dis. 1991; 35:356–359.


4. Breytenbach JH. Vaccination and biosecurity is the key. Poult World. 2005; 159:33.
5. Capua I, Mutinelli F, Marangon S, Alexander DJ. H7N1 avian influenza in Italy (1999 to 2000) in intensively reared chickens and turkeys. Avian Pathol. 2000; 29:537–543.


6. Chen H, Subbarao K, Swayne D, Chen Q, Lu X, Katz J, Cox N, Matsuoka Y. Generation and evaluation of a high-growth reassortant H9N2 influenza A virus as a pandemic vaccine candidate. Vaccine. 2003; 21:1974–1979.


7. Choi JG, Lee YJ, Kim YJ, Lee EK, Jeong OM, Sung HW, Kim JH, Kwon JH. An inactivated vaccine to control the current H9N2 low pathogenic avian influenza in Korea. J Vet Sci. 2008; 9:67–74.


8. Crinion RAP. Egg quality and production following infectious bronchitis virus exposure at one day old. Poult Sci. 1972; 51:582–585.


9. Crinion RAP, Ball RA, Hofstad MS. Abnormalities in laying chickens following exposure to infectious bronchitis virus at one day old. Avian Dis. 1971; 15:42–48.


10. De BK, Shaw MW, Rota PA, Harmon MW, Esposito JJ, Rott R, Cox NJ, Kendal AP. Protection against virulent H5 avian influenza virus infection in chickens by an inactivated vaccine produced with recombinant vaccinia virus. Vaccine. 1988; 6:257–261.


11. Di Trani L, Cordioli P, Falcone E, Lombardi G, Moreno A, Sala G, Tollis M. Standardization of an inactivated H17N1 avian influenza vaccine and efficacy against A/Chicken/Italy/13474/99 high-pathogenicity virus infection. Avian Dis. 2003; 47:Suppl 3. 1042–1046.


12. Hitchner SB. Virus propagation in embryonating eggs. In : Hitchner SB, Domermuth CH, Purchase HG, Williams JE, editors. Isolation and Identification of Avian Pathogens. 2nd ed. College Station: American Association of Avian Pathologists;1980. p. 120–121.
13. Karunakaran D, Newman JA, Halvorson DA, Abraham A. Evaluation of inactivated influenza vaccines in market turkeys. Avian Dis. 1987; 31:498–503.


14. Lee CH, Byun SH, Lee YJ, Mo IP. Genetic evolution of the H9N2 avian influenza virus in Korean poultry farms. Virus Genes. 2012; 45:38–47.


15. Lee CW, Song CS, Lee YJ, Mo IP, Garcia M, Suarez DL, Kim SJ. Sequence analysis of the hemagglutinin gene of H9N2 Korean avian influenza viruses and assessment of the pathogenic potential of isolate MS96. Avian Dis. 2000; 44:527–535.


16. Lee DH, Song CS. H9N2 avian influenza virus in Korea: evolution and vaccination. Clin Exp Vaccine Res. 2013; 2:26–33.


17. Lee HJ, Kwon JS, Lee DH, Lee YN, Youn HN, Lee YJ, Kim MC, Jeong OM, Kang HM, Kwon JH, Lee JB, Park SY, Choi IS, Song CS. Continuing evolution and interspecies transmission of influenza viruses in live bird markets in Korea. Avian Dis. 2010; 54:Suppl 1. 738–748.


18. Lu H, Castro AE, Pennick K, Liu J, Yang Q, Dunn P, Weinstock D, Henzler D. Survival of avian influenza virus H7N2 in SPF chickens and their environments. Avian Dis. 2003; 47:Suppl 3. 1015–1021.


19. Lu X, Edwards LE, Desheva JA, Nguyen DC, Rekstin A, Stephenson I, Szretter K, Cox NJ, Rudenko LG, Klimov A, Katz JM. Cross-protective immunity in mice induced by live-attenuated or inactivated vaccines against highly pathogenic influenza A (H5N1) viruses. Vaccine. 2006; 24:6588–6593.


20. Marangon S, Bortolotti L, Capua I, Bettio M, Dalla Pozza M. Low-pathogenicity avian influenza (LPAI) in Italy (2000-01): epidemiology and control. Avian Dis. 2003; 47:Suppl 3. 1006–1009.


21. Mo IP, Song CS, Kim KS, Rhee JC. An occurrence of non-highly pathogenic avian influenza in Korea. In : Proceedings of the Forth International Symposium on Avian Influenza; 29-31 May 1997; Tallahassee, USA. US Animal Health Association;p. 379–383.
22. Nili H, Asasi K. Natural cases and an experimental study of H9N2 avian influenza in commercial broiler chickens of Iran. Avian Pathol. 2002; 31:247–252.


23. North MO, Bell DD. Commercial Chicken Production Manual. 4th ed. New York: Springer US;1990. p. 433–452.
24. Skeeles JK, Beasley JN, Blore P, Klopp S. Severe egg-production drops in turkey breeders in southcentral Missouri. Avian Dis. 1981; 25:764–767.


25. Smyth JA, Platten MA, McFerran JB. A study of the pathogenesis of egg drop syndrome in laying hens. Avian Pathol. 1988; 17:653–666.


26. Swayne DE, Beck JR, Perdue ML, Beard CW. Efficacy of vaccines in chickens against highly pathogenic Hong Kong H5N1 avian influenza. Avian Dis. 2001; 45:355–365.


27. Swayne DE, Garcia M, Beck JR, Kinney N, Suarez DL. Protection against diverse highly pathogenic H5 avian influenza viruses in chickens immunized with a recombinant fowlpox vaccine containing an H5 avian influenza hemagglutinin gene insert. Vaccine. 2000; 18:1088–1095.


28. Tumpey TM, Kapczynski DR, Swayne DE. Comparative susceptibility of chickens and turkeys to avian influenza A H7N2 virus infection and protective efficacy of a commercial avian influenza H7N2 virus vaccine. Avian Dis. 2004; 48:167–176.


29. Van Eck JH, Davelaar FG, Van Den Heuvel-Plesman TAM, Van Kol N, Kouwenhoven B, Guldie FHM. Dropped egg production, soft shelled and shell-less eggs associated with appearance of precipitins to adenovirus in flocks of laying fowls. Avian Pathol. 1976; 5:261–272.

