Abstract
Cows infected with Escherichia (E.) coli usually experience severe clinical symptoms, including damage to mammary tissues, reduced milk yield, and altered milk composition. In order to investigate the host response to E. coli infection and discover novel markers for mastitis treatment, mammary tissue samples were collected from healthy cows and bovines with naturally occurring severe E. coli mastitis. Changes of mammary tissue proteins were examined using two-dimensional gel electrophoresis and label-free proteomic approaches. A total of 95 differentially expressed proteins were identified. Of these, 56 proteins were categorized according to molecular function, cellular component, and biological processes. The most frequent biological processes influenced by the proteins were response to stress, transport, and establishment of localization. Furthermore, a network analysis of the proteins with altered expression in mammary tissues demonstrated that these factors are predominantly involved with binding and structural molecule activities. Vimentin and α-enolase were central "functional hubs" in the network. Based on results from the present study, disease-induced alterations of protein expression in mammary glands and potential markers for the effective treatment of E. coli mastitis were identified. These data have also helped elucidate defense mechanisms that protect the mammary glands and promote the pathogenesis of E. coli mastitis.
Escherichia (E.) coli mastitis is a common disease in dairy cattle that is most frequently observed during early lactation, and infected animals exhibit a wide range of disease severity [8]. After intramammary inflammation, the milk composition changes, resulting in poor milk quality, increased whey protein, and decreased casein levels associated with elevated proteolytic activity [51331]. Additionally, the levels of various components of the host defense system including inflammatory cytokines, such as interleukin (IL)-1β, interferon-γ (INF-γ), IL-12, and transforming growth factor-α (TGF-α), along with anti-bacterial proteins, including cathelicidin 1, lactoperoxidase, and lactoferrin, increase in milk [1331]. These changes are mainly caused by intramammary inflammation and damage to the mammary gland.
ammary tissues of cows infected with mastitis have been reported. At the transcriptional level, individual gene and global gene-expression analyses have been conducted to study the effects of intramammary inflammation [712]. In particular, transcriptional analyses of uninfected mammary gland quarters, neighboring uninfected quarters, and quarters infected with the mastitis pathogens E. coli and Staphylococcus (S.) aureus have been performed. The results demonstrated that changes in transcription are principally associated with immune response functions [71215]. When evaluating proteins as chief actors within the cell, some groups have focused on examining the changes of mammary proteins from cows infected with mastitis. For example, psoriasin, a skin-resident anti-bacterial chemotactic protein belonging to the S100 family, was detected in the teat cistern of E. coli-infected cows with immunoblotting and immunohistochemistry [26]. Granulocyte chemotactic protein-2 and IL-8 were found to be expressed in mammary epithelial cell lines and neutrophils in response to exposure to lipopolysaccharides [38]. In particular, proteomics has been used to investigate mammary cancer cases [1035], liver health, and changes in the mammary gland during lactation [25]. Previously, we compared changes in mammary proteins between healthy and mastitic cows using a two-dimensional gel electrophoresis (2-DE) approach [37]. However, a proteomic analysis of mammary proteins has not been performed to evaluate host defense responses.
Proteomic approaches allow us to determine the whole-protein profiles of mammary tissue from cows infected with mastitis and detect any proteins affected by intramammary inflammation. We hypothesized that comparison of mammary proteins from cows with naturally occurring E. coli mastitis and healthy cows using a proteomics-based analysis would provide novel information. Thus, the objective of this investigation was to evaluate changes in mammary tissue from cows with severe mastitis compared to healthy bovines using 2-DE-based and label-free quantitative proteomics approaches. The results may provide promising information that will allow us to better understand the host defense mechanisms and discover molecular markers for treating mastitis.
Mammary tissue samples were obtained from the cistern tissues of slaughtered cows. Two cm or more of the mammary epithelium were collected from three healthy and mastitic cows from the Anhui area (China). The cows with E. coli mastitis were diagnosed according to visibly abnormal milk (e.g., clots, flakes, or watery texture) accompanied by swelling in the affected mammary quarter [13]. This diagnosis was made based on bacteriological culture of the milk on blood agar for all organisms along with McConkey and EMB agar for E. coli. The samples were also subjected to Gram staining and microscopy evaluation [29]. The healthy cows were identified based on somatic cell counts less than 200,000 cells/mL in milk measured using a Fossomatic 5000 instrument (Foss, Denmark). All samples were washed with PBS to remove the milk and debris, and then stored in liquid nitrogen.
The mammary tissues were manually homogenized with a mortar and pestle in liquid nitrogen. The resulting powder was transferred into a tube, three volumes of lysis solution (8 mol/L urea, 2 mol/L thiourea, 4% 3-[(3-cholamido-propy1)-dimethylammonio]-I-propanesulfonate, 40 mmol/L Tris, 65 mmol/L dithiotreitol [DTT], and 2% immobilized pH gradient [IPG] buffer) were added, and the solution was mixed for 20 min with periodic vortexing. Subsequently, the homogenate was centrifuged at 14,000 × g for 15 min at 4℃. The supernatant was then collected, and the protein concentrations were measured with a modified Bradford protein assay. The protein samples were stored at -80℃ until analysis.
Mammary tissue proteins (800 µg) were diluted in 350 µL of rehydration solution (8 mol/L urea, 2 mol/L thiourea, 4% 3-[(3-cholamido-propy1)-dimethylammonio]-I-propanesulfon ate, 40 mmol/L Tris, 65 mmol/L DTT, and 2% IPG buffer). Next, IPG strips (pH 3-10) 17 cm in length were used to separate the proteins in the first dimension using a Protean IEF cell (Bio-Rad Laboratories, USA) according to the manufacturer's instructions. After focusing, the IPG strips were placed in an equilibration solution (6 mol/L urea, 2% SDS, 20% glycerol, 50 mmol/L Tris-HCl, and 0.01% bromophenol blue) containing 2% DTT and 2.5% iodoacetamide for 12 min with shaking. For the second dimension, the strips were transferred to 12% SDS-PAGE gels and attached to the gel surface with 0.5% low-melting point agarose. The proteins were separated in a Protean II XI instrument (Bio-Rad Laboratories) at 50 V for 30 min and then at 200 V for 5 h.
The gels were incubated in a solution with 40% ethanol and 10% acetic acid for at least 3 h, and then stained with colloidal Coomassie blue G-250 solution [9]. Triplicate gels were run for each sample. The stained gels were scanned using a GS800 calibrated densitometer (Bio-Rad Laboratories) with 256 grayscale and 600-dpi parameters. Spot detection was performed using PDQuest 8.0.1 software (Bio-Rad Laboratories). To calculate the corrected protein intensities, the background values were removed and resulting intensities were then plotted as a function of the protein concentration. Protein spots that exhibited at least a two-fold decrease or increase in staining intensity were selected for further evaluation.
The selected protein spots were excised, transferred to tubes, and washed twice with MilliQ water for 15 min. Subsequently, the gel pieces were destained with 50% acetonitrile in 0.1 mol/L ammonium bicarbonate for 15 min at room temperature and dehydrated in acetonitrile. The dried gel pieces were then rehydrated in 0.01% sequence-grade trypsin (Promega, USA) and incubated at 37℃ for 16 h. The digestion was terminated by the addition of 1% trifluoroacetic acid.
The extracted peptides were loaded onto an AnchorChip plate for mass spectrometry analysis. Peptide mass spectra were obtained using an Ultraflex MALDI TOF/TOF mass spectrometer (Bruker Daltonics, Germany). The most prominent tryptic peptides were subjected to MS/MS analysis. The spectra were processed using FlexAnalysis 2.2 software tools (Bruker Daltonics). Protein identification was performed using MASCOT software to search the National Center for Biotechnology Information (NCBI, USA) non-redundant protein database (http://www.ncbi.nlm.nih.gov). Critical parameters included the following: monoisotopic mass accuracy, < 100 ppm; missed cleavages, 1; minimum signal-to-noise (S/N) ratio, 15; carbamidomethylation of cysteine as a fixed modification; MS/MS mode, and fragment mass tolerance, < 0.5 Da.
The powdered mammary tissues were dissolved in a solution of 8 mol/L urea and 2 mol/L thiourea. The supernatant was collected after centrifugation at 12,000 × g for 30 min at 4℃. The protein concentration was measured using a modified Bradford protein assay. The protein samples (100 µg) were reduced with 10 mmol/L DTT at 37℃ for 2.5 h and then alkylated with 50 mmol/L iodoacetamide at room temperature for 40 min. Next, the proteins were digested with sequencing grade-modified trypsin (Promega) using an enzyme-to-protein ratio of 1 : 50 at 37℃ for 20 h.
An Ettan MDLC system (GE Healthcare, UK) was used to desalt with Zorbax 300 SB C18 columns (Agilent Technologies, USA) and separate on a reverse phase column. Mobile phase A contained 0.1% formic acid in MilliQ water and mobile phase B consisted of 0.1% formic acid in acetonitrile. Approximately 20 µg of the trypsinized peptide mixture was loaded onto the column. Desalting and separation were initiated using 3% buffer B for 5 min, 4% to 50% buffer B for 90 min, 50% to 90% buffer B for 10 min, and 98% buffer A for 10 min at a flow rate of 200 nL/min. An electrospray ionization ion trap mass spectrometer (LTQ; Thermo Scientific, USA) was connected to the liquid chromatography apparatus to detect the eluted peptides. The MS/MS spectra were identified in the data-dependent mode. Each scan cycle included a full MS scan in profile mode and five MS/MS scans in centroid mode. Dynamic exclusion was set to a repeat count of 2, a repeat duration of 30 sec, and an exclusion duration of 90 sec. The peptides were identified using SEQUEST software (BioWorks 2.0; Thermo Electron, USA) to search the NCBI non-redundant protein database. The SEQUEST search parameters were based on Delta CN ≥ 0.1 along with Xcorr values of 1.9, 2.2, and 3.75 for 1+, 2+, and 3+ charged ions, respectively. All proteins with more than one unique identification were considered for protein quantification using spectral counting. The spectral count of each protein was calculated as the number of .dta files assigned to any peptide from the protein with high SEQUEST scores that fulfilled the DTASelect filtering criteria. The fold-change of protein expression was determined using a published formula for calculating spectral counting ratios [20].
Thirty µg of mammary proteins from control and mastitic cows were separated by 12% SDS-PAGE for 1 h, and then transferred onto a polyvinylidene difluoride (PVDF) membrane using a wet transfer apparatus (Bio-Rad Laboratories). Next, the PVDF membrane was blocked with 3% chicken serum and incubated with anti-bovine β-casein monoclonal antibodies (1 : 1,000) at 37℃ for 1 h. After washing, the membrane was incubated with horseradish peroxidase-coupled secondary antibody (1 : 5,000) for 1 h. Antibody binding was detected using diaminobenzidine as the substrate. The anti-bovine β-casein monoclonal antibodies were prepared by the Beijing Protein Institute (China).
Significance differences in staining intensities for mammary proteins from control cows and animals with severe E. coli mastitis according to 2-DE gel images and label-free mass spectrometry-based protein spectral counts were evaluated by t-tests using the Statistical Package of Social Science 16.0 (SPSS) program [22]. P values less than 0.05 were considered significant. A protein-protein interaction network of the differentially expressed proteins in mammary tissues, including the 2-DE and label-free data, was established by downloading the pathway data from the Kyoto Encyclopedia of Genes and Genomes (KEGG) database. Enzyme-enzyme and protein-protein interactions were then analyzed with the KEGGSOAP package of R software. The protein-protein network was identified and graphically visualized as nodes (proteins) and edges (relationships between proteins) using Medusa software [14].
2-DE and label-free-based proteomic approaches were used to compare changes in mammary tissue proteins between healthy cows and bovines with severe E. coli mastitis. Representative 2-DE gels of mammary tissue proteomes from the control and E. coli mastitic samples are presented in Fig. 1. A total of 14 proteins were downregulated while 12 proteins were upregulated in mammary tissues from cows with severe E. coli mastitis. Spots corresponding to these proteins were then subjected to mass spectrometry for identification. The levels of cofilin-1, αs1- and β-casein, serotransferrin, and fatty acid-binding protein were decreased whereas expression of inflammatory mediators and host defense proteins, including manganous superoxide dismutase, cathelicidin-1, S100 calcium-binding protein A11 (S100A11), S100 calcium-binding protein A12 (S100 A12), and S100 calcium-binding protein A8 (S100A8), were increased in cows with mastitis (Table. 1).
The label-free results revealed that the levels of 86 proteins were changed in mammary tissues from cows with E. coli mastitis (Table 2). Of these, 12 proteins (such as ATP synthase subunit alpha, fatty acid synthase, and isocitrate dehydrogenase) were only detected in mammary tissues of healthy cows while 45 proteins (including cathelicidin-1, galectin-1, and S100 A9) were only detected in mammary tissues of mastitic cows. Expression of the calcium uniporter channel components, galaptin, and vimentin was significantly increased whereas the levels of fatty-acid-binding protein, αs1-casein, and β-casein were decreased in cows with severe E. coli mastitis. Our proteomics result showing the downregulation of β-casein was validated by Western blot analysis (Fig. 2).
The differentially expressed proteins in mammary tissues identified through 2-DE and label-free methods were categorized based on their molecular function, cellular components, and biological processes using gene ontology (GO) annotations. The 56 identified proteins were assigned to each category as listed in Fig. 3. When molecular functions were evaluated, the highest enrichment was found for enzyme regulatory activities that included ones involving structural molecules, enzyme inhibitors, transporters, peptidase inhibitors, antioxidants, and hydro-lyases. Another major functional category was binding, which included binding to calcium ions, proteins, oxygen, and the cell surface. Analysis of the cellular component categories revealed that the highest enrichment was in the intracellular, intracellular organelle, and intracellular categories. Identification of biological processes influenced by the proteins showed that the most common processes were response to stress, transport, and establishment of localization.
To investigate the relationship between the differentially expressed proteins, a network analysis was performed using the KEGGSOAP package. Known interactions obtained from the KEGG database were visualized as nodes and edges. The number of relationships for each protein was calculated. The network map and degree of relationship for each identified proteins are depicted in Fig. 4. Interestingly, most of the proteins in the network were involved in binding and structural molecule activity. Vimentin and α-enolase were central "functional hubs" in the map (i.e., these factors were involved in more relationships than the other proteins).
In the current study, differentially expressed proteins in mammary tissues of healthy cows and cows with severe E. coli mastitis were identified using 2-DE and label-free proteomics approaches. The proteomics results for β-casein were confirmed by Western blot analyses. In addition, a protein interaction network of the differentially expressed proteins was constructed, and demonstrated that vimentin and α-enolase act as central "functional hubs" in the network.
In our investigation, the levels of caseins (αs1-, αs2-, and β-casein) were decreased in mammary tissues of cows with severe E. coli mastitis. This result is due to reduced protein synthesis caused by DNA-remethylation around a signal transducer and activator of transcription 5 (STAT5)-binding enhancer in the αs1-casein promoter as was observed in a previous study [34]. Additionally, numerous studies have shown that the concentration of caseins is decreased in milk from cows infected with mastitis [61333].
Our results revealed that the levels of several families of antimicrobial proteins were elevated in the mammary tissue of cows with severe E. coli mastitis. For instance, several members of the cathelicidin family of proteins (cathelicidin 1, 2, and 4) that were increased in cows with severe mastitis form a family of cationic antimicrobial peptides that perform a wide spectrum of antimicrobial activities [39]. Cathelicidins were also found to be upregulated in milk samples from mastitic cows in previous studies [2730]. These results suggest that cathelicidins can eliminate mastitis pathogens and protect the mammary glands.
Our data showed that S100 A11, S100 A2, S100 A9, S100 A12, and S100 A8 were upregulated in the mammary gland of cows with mastitis. These proteins are Ca2+-binding proteins belonging to the S100 multigenic family that have been shown to have antimicrobial activities associated with innate immunity, and are predominantly produced by neutrophils, monocytes, and activated macrophages [21]. A recent study demonstrated that S100 A12 expression is significantly increased in milk from cows subjected to intra-mammary challenge with E. coli [5]. This increase is the result of enhanced S100 A12 expression in bovine mammary tissue in response to the challenge [17]. According to our results, the increased levels of antimicrobial proteins corresponded to a reduction in the relative abundance of caseins in the mammary gland of cows infected with mastitis, indicating that a defense response to infections is mounted in the mammary gland of cows.
More notably, the levels of cytoskeletal-associated proteins, such as thymosin β-4, peripherin, vimentin, cytokeratin-14, and keratin 13, were significantly increased in cows with severe mastitis. Thymosin β-4, a multi-functional regenerative peptide, is specifically produced and released by the thymic gland, and possesses hormonal activities that modulate the immune response [18], regulate intracellular signal transduction, and affect the cytoskeletal structure [28]. In addition, thymosin β-4 plays a vital role in wound repair as well as the regeneration of injured cells and tissues by decreasing the number of myofibroblasts in wounds to reduce scar formation and fibrosis [1123]. In the current study, thymosin β-4 levels were elevated in cows with severe E. coli mastitis. This protein has been used to protect cells and tissues from damage while reducing apoptosis, inflammation, and microbial growth [24]. Peripherin is a type III intermediate filament protein that is widely expressed in the cell body and axons of neurons in the peripheral nervous system. Numerous studies have demonstrated that peripherin expression is upregulated during neuronal injury and mediates neuronal repair [1936]. This factor serves as a highly specialized cytoskeletal stress protein that promotes cellular organization and homeostasis [32]. In the current study, some cytoskeleton-associated proteins that exhibited increased expression in cows with severe E. coli mastitis have cytoprotective functions, including the inhibition of apoptosis, organelle homeostasis, and scaffolding.
We further determined that vimentin and α-enolase are central "functional hubs" in the protein-protein interaction network we generated because these factors were involved in more relationships than the other proteins. Vimentin is the intermediate filament protein normally expressed in mammary epithelial cells and has been used to evaluate primary cultures of mammary epithelial cells [2]. Little information is available about the relationship between intramammary infection and vimentin. In addition, α-enolase, a non-classical plasminogen-binding protein and a blood coagulation factor, has been found in bovine neutrophils and bacteria [416] The levels of both vimentin and α-enolase were increased in the mammary glands of cows with severe E. coli mastitis in the present study, and may serve as effector proteins for the immune defense or facilitate pathogen invasion. These results require further investigation.
In summary, changes of mammary proteins in healthy cows compared to cows with E. coli mastitis were investigated using proteomic approaches. Results of a protein-protein network analysis indicated that the differentially expressed proteins are associated with binding and structural molecule activities in cows infected with severe E. coli mastitis. Additionally, vimentin and α-enolase act as central "functional hubs" in this network. Based on our findings, potential effective markers may be identified for the treatment of mastitis and foster a better understanding of mechanisms underlying the host defense system activated in response to E. coli mastitis.
Figures and Tables
Fig. 1
Changes in the protein spots detected on two-dimensional gel electrophoresis (2-DE) gels of mammary tissues from healthy cows and animals with Escherichia (E.) coli mastitis. (A) 2-DE gel for mammary tissues from healthy cows. (B) 2-DE gel for mammary tissues from cows with severe E. coli mastitis. The labels and arrows indicating spots corresponding to proteins with altered expression levels.
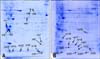
Fig. 2
Abundance of β-casein in mammary tissues from cows with severe E. coli mastitis and healthy animals. (A) Representative images of spots corresponding to β-casein in the 2-DE map. (B) Densitometric analysis of the β-casein spots in the 2-DE map. (C) Western blot analysis of β-casein expression. (D) Densitometric analysis of the Western blot. Values are presented as the mean ± standard error (SE). *p < 0.05 (n = 3).
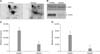
Fig. 3
Classification of differentially expressed proteins in mammary tissues from healthy cows and bovines with E. coli mastitis based on gene ontology annotations. (A) Molecular function. (B) Cellular component. (C) Biological process.
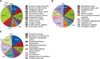
Fig. 4
Protein-protein network of differentially expressed proteins in mammary tissues from control cows and cows with E. coli mastitis. (A) Graphic depiction of the degree of connections for each protein in the network. (B) Representative image of the protein-protein interaction network. Pink lines indicate the connections confirmed by the experimental study, the blue lines indicate connections derived from the databases, and the yellow lines indicate connections described in abstracts of articles published in the literature.
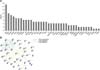
Table 1
Spots corresponding to proteins with altered expression in mammary tissues from healthy cows versus cows with severe E. coli mastitis as identified by MALDI TOF/TOF MS
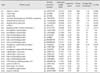
*Negative values indicate decreased expression in cows with E. coli mastitis, positive values correspond to increased expression in animals with E. coli mastitis, "D" indicates that the protein was detected only in healthy cows, and "U" means that the protein was detected only in animals with E. coli mastitis. NA, not available.
Acknowledgments
The project was supported by the Anhui Academy of Agricultural Sciences (project no. 14C0403), and the Scientific and Technological Achievements from the Ministry of Science and Technology (project no. 2013GB2C300216), China.
References
1. Alonso-Fauste I, Andrés M, Iturralde M, Lampreave F, Gallart J, Alava MA. Proteomic characterization by 2-DE in bovine serum and whey from healthy and mastitis affected farm animals. J Proteomics. 2012; 75:3015–3030.


2. Anaya-López JL, Contreras-Guzmán OE, Cárabez-Trejo A, Baizabal-Aguirre VM, López-Meza JE, Valdez-Alarcón JJ, Ochoa-Zarzosa A. Invasive potential of bacterial isolates associated with subclinical bovine mastitis. Res Vet Sci. 2006; 81:358–361.


3. Bannerman DD, Kauf AC, Paape MJ, Springer HR, Goff JP. Comparison of Holstein and Jersey innate immune responses to Escherichia coli intramammary infection. J Dairy Sci. 2008; 91:2225–2235.


4. Boehmer JL. Proteomic analyses of host and pathogen responses during bovine mastitis. J Mammary Gland Biol Neoplasia. 2011; 16:323–338.


5. Boehmer JL, Bannerman DD, Shefcheck K, Ward JL. Proteomic analysis of differentially expressed proteins in bovine milk during experimentally induced Escherichia coli mastitis. J Dairy Sci. 2008; 91:4206–4218.


6. Boehmer JL, Ward JL, Peters RR, Shefcheck KJ, McFarland MA, Bannerman DD. Proteomic analysis of the temporal expression of bovine milk proteins during coliform mastitis and label-free relative quantification. J Dairy Sci. 2010; 93:593–603.


7. Buitenhuis B, Røntved CM, Edwards SM, Ingvartsen KL, Sørensen P. In depth analysis of genes and pathways of the mammary gland involved in the pathogenesis of bovine Escherichia coli-mastitis. BMC Genomics. 2011; 12:130.
8. Burvenich C, Van Merris V, Mehrzad J, Diez-Fraile A, Duchateau L. Severity of E. coli mastitis is mainly determined by cow factors. Vet Res. 2003; 34:521–564.
9. Candiano G, Bruschi M, Musante L, Santucci L, Ghiggeri GM, Carnemolla B, Orecchia P, Zardi L, Righetti PG. Blue silver: a very sensitive colloidal Coomassie G-250 staining for proteome analysis. Electrophoresis. 2004; 25:1327–1333.


10. Fink-Retter A, Czerwenka K, Gschwantler-Kaulich D, Hudelist G, Pischinger K, Manavi M, Kubista E, Singer CF. Proteomics in mammary cancer research. Eur J Gynaecol Oncol. 2009; 30:635–639.


11. Goldstein AL, Hannappel E, Sosne G, Kleinman HK. Thymosin β4: a multi-functional regenerative peptide. Basic properties and clinical applications. Expert Opin Biol Ther. 2012; 12:37–51.


12. Hiss S, Mielenz M, Bruckmaier RM, Sauerwein H. Haptoglobin concentrations in blood and milk after endotoxin challenge and quantification of mammary Hp mRNA expression. J Dairy Sci. 2004; 87:3778–3784.


13. Hogarth CJ, Fitzpatrick JL, Nolan AM, Young FJ, Pitt A, Eckersall PD. Differential protein composition of bovine whey: a comparison of whey from healthy animals and from those with clinical mastitis. Proteomics. 2004; 4:2094–2100.


14. Hooper SD, Bork P. Medusa: a simple tool for interaction graph analysis. Bioinformatics. 2005; 21:4432–4433.


15. Jensen K, Günther J, Talbot R, Petzl W, Zerbe H, Schuberth HJ, Seyfert HM, Glass EJ. Escherichia coli- and Staphylococcus aureus-induced mastitis differentially modulate transcriptional responses in neighbouring uninfected bovine mammary gland quarters. BMC Genomics. 2013; 14:36.


16. Lippolis JD, Reinhardt TA. Proteomic survey of bovine neutrophils. Vet Immunol Immunopathol. 2005; 103:53–65.


17. Lutzow YCS, Donaldson L, Gray CP, Vuocolo T, Pearson RD, Reverter A, Byrne KA, Sheehy PA, Windon R, Tellam RL. Identification of immune genes and proteins involved in the response of bovine mammary tissue to Staphylococcus aureus infection. BMC Vet Res. 2008; 4:18.
18. Mannherz HG, Hannappel E. The β-thymosins: intracellular and extracellular activities of a versatile actin binding protein family. Cell Motil Cytoskeleton. 2009; 66:839–851.


19. McLean J, Liu HN, Miletic D, Weng YC, Rogaeva E, Zinman L, Kriz J, Robertson J. Distinct biochemical signatures characterize peripherin isoform expression in both traumatic neuronal injury and motor neuron disease. J Neurochem. 2010; 114:1177–1192.


20. Old WM, Meyer-Arendt K, Aveline-Wolf L, Pierce KG, Mendoza A, Sevinsky JR, Resing KA, Ahn NG. Comparison of label-free methods for quantifying human proteins by shotgun proteomics. Mol Cell Proteomics. 2005; 4:1487–1502.


21. Perera C, McNeil HP, Geczy CL. S100 Calgranulins in inflammatory arthritis. Immunol Cell Biol. 2010; 88:41–49.


22. Pham TV, Piersma SR, Warmoes M, Jimenez CR. On the beta-binomial model for analysis of spectral count data in label-free tandem mass spectrometry-based proteomics. Bioinformatics. 2010; 26:363–369.


23. Philp D, Kleinman HK. Animal studies with thymosin β4, a multifunctional tissue repair and regeneration peptide. Ann N Y Acad Sci. 2010; 1194:81–86.


24. Qiu P, Wheater MK, Qiu Y, Sosne G. Thymosin β4 inhibits TNF-α-induced NF-κB activation, IL-8 expression, and the sensitizing effects by its partners PINCH-1 and ILK. FASEB J. 2011; 25:1815–1826.


25. Rawson P, Stockum C, Peng L, Manivannan B, Lehnert K, Ward HE, Berry SD, Davis SR, Snell RG, McLauchlan D, Jordan TW. Metabolic proteomics of the liver and mammary gland during lactation. J Proteomics. 2012; 75:4429–4435.


26. Regenhard P, Petzl W, Zerbe H, Sauerwein H. The antibacterial psoriasin is induced by E. coli infection in the bovine udder. Vet Microbiol. 2010; 143:293–298.


27. Reinhardt TA, Sacco RE, Nonnecke BJ, Lippolis JD. Bovine milk proteome: quantitative changes in normal milk exosomes, milk fat globule membranes and whey proteomes resulting from Staphylococcus aureus mastitis. J Proteomics. 2013; 82:141–154.


28. Ryu YK, Lee YS, Lee GH, Song KS, Kim YS, Moon EY. Regulation of glycogen synthase kinase-3 by thymosin beta-4 is associated with gastric cancer cell migration. Int J Cancer. 2012; 131:2067–2077.


29. Sears PM, González RN, Wilson DJ, Han HR. Procedures for mastitis diagnosis and control. Vet Clin North Am Food Anim Pract. 1993; 9:445–468.


30. Smolenski GA, Wieliczko RJ, Pryor SM, Broadhurst MK, Wheeler TT, Haigh BJ. The abundance of milk cathelicidin proteins during bovine mastitis. Vet Immunol Immunopathol. 2011; 143:125–130.


31. Suojala L, Orro T, Järvinen H, Saatsi J, Pyörälä S. Acute phase response in two consecutive experimentally induced E. coli intramammary infections in dairy cows. Acta Vet Scand. 2008; 50:18.
32. Toivola D, Strnad P, Habtezion A, Omary M. Intermediate filaments take the heat as stress proteins. Trends Cell Biol. 2010; 20:79–91.


33. Urech E, Puhan Z, Schällibaum M. Changes in milk protein fraction as affected by subclinical mastitis. J Dairy Sci. 1999; 82:2402–2411.


34. Vanselow J, Yang W, Herrmann J, Zerbe H, Schuberth HJ, Petzl W, Tomek W, Seyfert HM. DNA-remethylation around a STAT5-binding enhancer in the αS1-casein promoter is associated with abrupt shutdown of αS1-casein synthesis during acute mastitis. J Mol Endocrinol. 2006; 37:463–477.


35. Warmoes M, Jaspers JE, Pham TV, Piersma SR, Oudgenoeg G, Massink MP, Waisfisz Q, Rottenberg S, Boven E, Jonkers J, Jimenez CR. Proteomics of mouse BRCA1-deficient mammary tumors identifies DNA repair proteins withcancer. Mol Cell Proteomics. 2012; 11:M111.013334.
36. Xiao S, Tjostheim S, Sanelli T, McLean JR, Horne P, Fan Y, Ravits J, Strong MJ, Robertson J. An aggregate-inducing peripherin isoform generated through intron retention is upregulated in amyotrophic lateral sclerosis and associated with disease pathology. J Neurosci. 2008; 28:1833–1840.


37. Yang Y, Zhao X, Zhang Y. Proteomic analysis of mammary tissues from healthy cows and clinical mastitic cows for identification of disease-related proteins. Vet Res Commun. 2009; 33:295–303.

