Abstract
Salmonella enterica Gallinarum (SG) causes fowl typhoid (FT), a septicemic disease in avian species. We constructed deletion mutants lacking the stress sigma factor RpoS, the nitric oxide (NO)-detoxifying flavohemoglobin Hmp, and the SsrA/SsrB regulator to confirm the functions of these factors in SG. All gene products were fully functional in wild-type (WT) SG whereas mutants harboring single mutations or a combination of rpoS, hmp, and ssrAB mutations showed hypersusceptibility to H2O2, loss of NO metabolism, and absence of Salmonella pathogenicity island (SPI)-2 expression, respectively. A triple-deletion mutant, SGΔ3 (SGΔrpoSΔhmpΔssrAB), was evaluated for attenuated virulence and protection efficacy in two-week-old Lohmann layer chickens. The SGΔ3 mutant did not cause any mortality after inoculation with either 1 × 106 or 1 × 108 colony-forming units (CFUs) of bacteria. Significantly lower numbers of salmonellae were recovered from the liver and spleen of chickens inoculated with the SGΔ3 mutant compared to chickens inoculated with WT SG. Vaccination with the SGΔ3 mutant conferred complete protection against challenge with virulent SG on the chickens comparable to the group vaccinated with a conventional vaccine strain, SG9R. Overall, these results indicate that SGΔ3 could be a promising candidate for a live Salmonella vaccine against FT.
Salmonella enterica serovar Gallinarum (SG) causes fowl typhoid (FT), a severe septicemic disease in poultry. This disease causes significant morbidity and mortality, and is a major concern in the poultry industry [3]. Over recent decades, attenuated SG strain 9 (SG9R) has been used as a live vaccine to control FT [3]. However, several limitations have been reported for this strain, including incomplete protection [4], virulence in certain breeds of chickens [22], a need for multiple injections to provide adequate protection [22], and virulence in newly hatched and young chicks [3]. Moreover, SG9R-like strains have been isolated from chicken field outbreaks [1624], and the genetic basis for attenuated virulence in SG9R is unknown [23]. For enhanced vaccine safety, it is therefore recommended that attenuated strains with well-defined genetics be explored.
Mutations of several genes that affect the intraphagosomal survival of Salmonella enterica serovar Typhimurium (ST) in host macrophages have been shown to attenuate the virulence of the bacterium. The rpoS gene (NC_003197.1) encodes the alternative sigma factor σs (RpoS) in Salmonella. RpoS promotes adaptation to a range of stress conditions and starvation. It also contributes to Salmonella virulence via regulation of the virulence plasmid gene spv and chromosomal genes [115]. Mutations of the rpoS gene attenuate the virulence of ST [9], and it was reported that rpoS mutants confer high levels of protection against a virulent ST strain in mice [6].
The hmp (NC_016857.1) gene encodes the flavohemoglobin Hmp in Salmonella. Hmp provides the principal means of protection of Salmonella from nitric oxide (NO) and nitrosative stress within professional phagocytes [11]. An ST mutant lacking this enzyme is hypersensitive to nitrosative stress, accumulates S-nitrosylated proteins in its cytoplasm, and shows attenuated virulence in mice [2]. The virulence genes located on Salmonella pathogenicity island (SPI)-2 encode a type III secretion system (T3SS) and a set of effector proteins that, through interaction with intracellular traffic in macrophages and dendritic cells, create a safe compartment for the salmonellae inside the phagocyte. A two-component regulatory system consisting of a sensor kinase (SsrA) and a response regulator (SsrB) responds to environmental signals [81725] and controls SPI-2 expression. Mutation of ssrAB (NC_003197.1) in ST and SG results in defective intracellular replication, and the virulence of ST mutant was reported to be attenuated in a mouse model [513].
It is important to note that the pathogenesis of Salmonella infection varies depending on the host-strain interaction [31819]. The roles of rpoS, hmp, and ssrAB in the pathogenesis of avian-specific SG have not been reported. Therefore, the objectives of the present study were: 1) construct deletion mutants SGΔrpoS, SGΔhmp, and SGΔssrAB, and validate the importance of these genes in SG; 2) construct a triple mutant, SGΔrpoSΔhmpΔssrAB (designated hereafter as SGΔ3), and investigate the effectiveness of this mutant as a live vaccine for FT prevention in chickens.
The bacterial strains and plasmids used in this study are listed in Table 1. SG97 was used as the parental SG strain. These bacteria were isolated from a layer chicken in Gangwon-do (Korea) in 2000, and the genetic type was identified by pulsed-field gel electrophoresis. The bacteria were grown at 37℃ with shaking in Luria-Bertani (LB) broth (Difco, USA) or minimal E medium (1.66 mM MgSO4, 9.5 mM citric acid monohydrate, 57 mM K2HPO4, and 16.7 mM NaNH3PO4) supplemented with 0.2% glucose (EG medium). The LB broth also contained 200 µg/mL ampicillin (AP), 50 µg/mL kanamycin (KM), and 20 µg/mL chloramphenicol (CM). S-nitrosoglutathione (GSNO) was synthesized via the reaction of glutathione and acidified sodium nitrite as previously described [112]. All chemicals were purchased from Sigma-Aldrich (USA) unless otherwise stated.
The SG mutants were constructed using the λ Red recombinase system [7]. The primers used to generate mutations in the SG cells are listed in Table 2. To generate the deletion mutants SGΔrpoS, SGΔhmp, and SGΔssrAB, the primer pairs rpoS-P1/rpoS-P2, hmp-P1/hmp-P2, and ssrA-P2/ssrB-P1, respectively, were used. The primer pairs rpoS-Fw/rpoS-Rev, hmp-Fw/hmp-Rev, and ssrA-Fw/ssrB-Rev were used to confirm the mutations by PCR. To construct the triple mutant SGΔ3, the chloramphenicol resistance cassette in the ssrAB::Cm mutant was first removed using flippase (FLP) recombinase encoded in the pCP20 plasmid, resulting in an ssrAB mutant lacking chloramphenicol resistance. The SGΔ3 mutants were then constructed by sequential transduction of rpoS::Km and hmp::Cm into the ssrAB mutant with removal of the antibiotic resistance cassette by FLP recombinase after each transduction.
Overnight cultures were added to the wells of a microtiter plate containing LB or EG medium (OD600 = 0.02). H2O2 was added to LB medium as an oxidative agent, and the NO congener GSNO was added to EG medium. Bacterial growth at 37℃ with shaking was monitored by measuring the OD600 at 30-min intervals.
The transcription of SPI-2 in SG was induced by reducing the extracellular Mg2+ concentration as previously described [8]. Briefly, Salmonella cells grown overnight in LB broth were washed once with high-Mg2+ N salts medium [5 mM KCl, 7.5 mM (NH4)2SO4, 0.5 mM K2SO4, 1 mM KH2PO4, 38 mM glycerol, 0.2% casamino acid, 10 mM MgCl2, and 100 mM Tris-HCl at pH 7.6], subcultured in fresh high-Mg2+ N salts medium, and grown at 37℃ to early log phase (OD600 = 0.5). Next, the bacterial cells were harvested, resuspended in a low-Mg2+ (8 µM MgCl2) N salts medium (pH 6.9) to induce SPI-2 expression or high-Mg2+ N salts medium as a control, and incubated for another 3 h. Transcription was stopped by adding a one-fifth volume of ice-cold phenol/ethanol (5% phenol in 95% ethanol) solution before harvesting the cells. Total RNA was extracted from the bacteria using RNAiso Plus reagent (Takara Bio, Japan). To measure the transcription levels of SPI-2, quantitative real-time RT-PCR was performed using a QuantiTect SYBR Green RT-PCR kit (Qiagen, Germany), as previously described [1]. DNA sequences of the primers used for this analysis are listed in Table 2.
Bacteria were grown in 10 mL LB broth at 37℃ with shaking to OD600~1.0. The harvested cells were washed once with phosphate-buffered saline (PBS) and resuspended in 10 mL PBS prewarmed to 37℃. The bacterial culture was continuously stirred with a magnetic stirring bar. The rate of NO consumption was determined by measuring the NO remaining after addition of 2 µM PROLI NONOate (Cayman Chemical, USA) to the bacterial solution. The concentration of NO in the solution was measured using a NO-sensitive electrode with a 2-mm diameter tip (ISO-NOP sensor; World Precision Instruments, USA) connected to a free radical analyzer (TBR4100; World Precision Instruments). The data signal was obtained using the LabChart program and expressed in picoamperes.
Three groups of 2-week-old Lohmann layer chickens were used for protocols approved by the Kangwon University Institutional Animal Care and Use Committee (permit no. KW-130924-2). To determine virulence of the triple mutant, groups of five chickens were housed separately and inoculated orally with 500 µL containing 1 × 106 or 1 × 108 colony-forming units (CFUs) of SGΔ3 or 500 µL containing 1 × 108 CFUs of wild-type (WT) SG. Each group of chickens was monitored for 2 weeks to measure mortality. At the end of each experiment, all the surviving chickens were humanely sacrificed, and the livers and spleens were collected. The tissue samples were homogenized with a tissue lyser (Qiagen) in peptone-buffered saline. Diluted homogenates were used to inoculate Shigella-Salmonella agar. After incubating at 37℃ for 24 h, the CFUs were counted.
To evaluate the protection conferred by the triple mutant against SG infection, 2-week-old Lohmann layer chickens were divided into five groups. Two groups (n = five birds in each group) were primed and boosted at 2 and 4 weeks of age either orally or subcutaneously (SC) with 500 µL of a suspension containing 1 × 108 CFUs of SGΔ3. Another two groups (n = five birds each group) were primed and boosted at 2 and 4 weeks of age either orally or SC with 500 µL of a suspension containing 1 × 108 CFUs of the SG9R vaccine strain. The negative control group was not vaccinated. At 6 weeks of age, all groups were challenged orally with 500 µL of a suspension containing 4 × 108 CFUs of WT SG. Mortality was assessed daily for 14 days post-challenge (dpc). All surviving chickens were euthanized at 14 dpc, and the livers and spleens were collected to measure the CFU counts.
To examine the role of RpoS in the resistance of SG to oxidative stress, we compared the growth of SGΔrpoS and SGΔ3 mutants to that of parental WT SG in LB media containing H2O2. As an uncharged molecule, H2O2 can freely diffuse through the bacterial cell membrane and damage various macromolecules. Because high iron levels further increase the cytotoxicity of H2O2 by producing hydroxyl radicals via Fenton chemistry, we used iron-rich LB media. Growth of the SG mutant strains lacking RpoS was temporally inhibited by H2O2 while that of the WT SG was slightly delayed (panel A in Fig. 1). When the SG genome was sequenced, it was found to contain a conserved katE gene encoding a catalase. Quantitative real-time RT-PCR analysis showed that katE transcription was reduced by 3- to 7-fold in SG strains containing the rpoS mutation (Fig. 2). These data clearly indicate that SG has conserved RpoS-dependent gene regulation that plays an important role in the antioxidant defense of the bacteria.
To evaluate the role of flavohemoglobin Hmp in the resistance of SG to nitrosative stress, we measured the NO consumption rate of WT and hmp mutant SG. After NO was added to the bacterial cultures, WT SG consumed almost all the NO within 1 min. In contrast, the hmp mutant strains barely metabolized NO, maintaining NO levels comparable to that of the buffer control. These data indicate that flavohemoglobin Hmp metabolizes almost all NO that enters into the SG cell (panel A in Fig. 3). Real-time RT-PCR showed that NO treatment increased the level of hmp transcription in WT SG up to 20-fold (panel B in Fig. 3), suggesting that SG has a regulatory system that controls Hmp expression in response to NO. Moreover, the growth rate of WT and hmp mutant SG in NO-producing cultures also indicated the importance of Hmp for resistance to nitrosative stress (panel B in Fig. 1). Collectively, these observations demonstrated that flavohemoglobin Hmp is required for SG defense against NO-mediated nitrosative stress.
To explore the regulation of SPI-2 gene expression in SG, we measured the transcription of sseJ and ssaB, which encode effector proteins, and sseA that encodes chaperones of the SPI-2 T3SS. Transcription levels of sseA, ssaB, and sseJ in SG cultures shifted from high to low Mg concentrations were 3.5, 6, and 5 times higher, respectively, compared to levels observed in SG cultured continuously grown in high-Mg media (Fig. 4). The transcription of sseA, ssaB, and sseJ was clearly abolished in the ssrAB mutant, demonstrating that existence of the SPI-2 T3SS in SG is subject to SsrA/SsrB-dependent regulation.
As shown in Fig. 1, the growth curves of SG mutants cultured without any additional stressors were very similar to that of WT SG. All strains showed a 2-h lag phase and reached a stationary phase within 12 h in LB broth. These results indicated that the growth of SGΔ3 was not defective. All mutants containing an rpoS mutation had almost the same level of susceptibility to H2O2. Additionally, all hmp mutants were unable to metabolize NO and showed the same susceptibility to nitrosative stress. Moreover, SsrAB-dependent SPI-2 gene regulation in SGΔ3 cells was comparable to that in the SGΔssrAB mutant. These data demonstrate that the phenotypes of each deletion mutant were observed in SGΔ3 cells.
Virulence testing was performed by inoculating Lohmann layer chickens orally with SGΔ3 and WT SG. One chicken infected with WT SG died 7 days post-inoculation while no deaths occurred in the SGΔ3 group. All the surviving birds were sacrificed 2 weeks post-inoculation, and the numbers of viable bacteria in the liver and spleen were determined (Fig. 5). The number of CFUs isolated from the liver and spleen of chickens inoculated with SGΔ3 (low and high doses) was significantly lower than that isolated from chickens inoculated with WT SG. Bacterial counts for the liver and spleen were also significantly different between chickens inoculated with low and high doses of SGΔ3. The mutant did not induce splenomegaly that was evident in the group inoculated with WT SG (data not shown). These results indicate that virulence of the SGΔ3 mutant was attenuated compared to WT SG.
Vaccinated chickens were challenged with SG and observed for 2 weeks. In nonvaccinated chickens, 60% mortality was observed from 7~14 dpc (Fig. 6). Chickens immunized (both SC and orally) with SGΔ3 and SG9R were completely protected from subsequent WT SG challenge. No deaths were observed in these groups. This finding suggests that the SGΔ3 mutant conferred a level of protection similar to that conferred by SG9R.
At 14 dpc, all the surviving chickens were sacrificed, and the SG counts for the liver and spleen were determined. In both organs, the number of CFUs in chickens vaccinated (either SC or orally) with SGΔ3 or SG9R was significantly lower than in the nonvaccinated group. However, the CFU counts for the SGΔ3-vaccinated groups were lower than those for the nonvaccinated group, but higher than that for the SG9R-vaccinated group.
In the present study, deletion mutants were constructed to determine the contribution of rpoS, hmp, and ssrAB to the pathogenesis of SG. We also evaluated the ability of the SGΔ3 mutant (SGΔrpoSΔhmpΔssrAB) to protect layer chickens against SG infection. Different Salmonella serovars have evolved to survive and adapt to host-specific niches through either the acquisition or loss of genes and gene functions (pseudogenes) [10]. Since different virulence factors are utilized depending on the host, pathogenesis and disease manifestation also vary among serovars. Thus, the functions of virulence genes cannot necessarily be extrapolated across different serovars. RpoS is known to act as an alternative sigma factor for genes associated with general stress resistance and virulence [15]. The katE gene (encoding catalase hydroperoxidase II [HPII]), which is involved in the resistance to H2O2, is under the control of RpoS. Consistent with previous reports, the SGΔ rpoS mutant in this study appeared susceptible to H2O2 stress [120]. Furthermore, real-time RT-PCR results revealed that the H2O2-sensitive phenotype resulting from the rpoS deletion was associated with a failure to induce the expression of KatE catalase. In host phagocytic cells, NO is generated by enzyme-inducible NO synthase. NO is a precursor for a range of reactive nitrogen species that have potent cytocidal and/or cytostatic activities against bacteria [11]. Flavohemoglobin Hmp has been reported to play a crucial role in the protection of ST against toxic effects of NO. As observed in a previous study on ST [2], the SGΔhmp mutant failed to decompose NO and its growth was severely impaired in NO-producing media compared to WT SG. Nitrosative stress also upregulated hmp expression in WT SG.
The SPI chromosomal regions encode important Salmonella virulence factors [25]. Among these, SPI-2 has been reported to be essential for the systemic virulence of Salmonella. The T3SS encoded by SPI-2 mediates the translocation of a large number of effector proteins into eukaryotic target cells. The translocated effector proteins modify normal host cell functions and permit intracellular Salmonella proliferation. Consistent with previous studies, findings from the present investigation showed that the expression of SPI-2 virulence genes in SG is regulated by ssrAB [51725]. Overall, these results establish the roles of rpoS, hmp, and ssrAB in the pathogenesis of SG infection.
To develop a highly attenuated and safe SG vaccine strain, an SGΔ3 mutant with three gene deletions (SGΔrpoSΔhmpΔssrAB) was constructed. This strain should 1) be highly susceptible to the toxic effects of oxidative and nitrosative stresses, 2) have defective production of virulence factors, 3) less likely than SG9R to revert to a virulent phenotype, and 4) be highly susceptible to various environmental stresses. SGΔ3 retained the defects observed for each individual gene deletion. Virulence of the SGΔ3 mutant was successfully attenuated as it was unable to cause mortality in chickens even when the birds were inoculated with 108 bacteria, and significantly fewer Salmonella CFUs were recovered from the liver and spleen compared to WT SG. This observation is in accordance with previous reports on the deletion of rpoS, hmp, and ssrAB genes in ST [2569]. In another previous study of a gene mutation affecting the structure of the SPI-2 T3SS (ΔssaU), failure to recover bacteria from the liver and spleen was reported [14]. The discrepant results observed were possibly due to 1) a difference in the virulence of SGΔssaU, 2) different breed of chicken used, 3) different age at the time of inoculation, or 4) a weaker effect of the ssrAB mutation on reducing SPI-2 expression than the combined effect of the mutations in individual genes of SPI-2. Immunization with SGΔ3 offered efficient in vivo protection against challenge with WT SG while 60% mortality was observed in the nonvaccinated group, indicating that SGΔ3 is an attractive live vaccine candidate. However, more studies on the safety, efficacy, and immunogenicity of the SGΔ3 mutant in different breeds and/or age groups of chickens are necessary.
In conclusion, in the present study we validated the importance of rpoS, hmp, and ssrAB in SG. The ability of the SGΔ3 mutant to confer protection against SG infection was evaluated in layer chickens. The results showed that SGΔ3 is attenuated and provides efficient protection against WT SG challenge.
Figures and Tables
Fig. 1
Susceptibility of SG strains to H2O2 or GSNO. Bacterial cells were (A) grown in microtiter plates containing LB broth in the presence (closed symbols) or absence (open symbols) of 250 µM H2O2, and (B) cultured in a microtiter plate containing EG media with (closed symbols) or without (open symbols) 1 mM GSNO. OD600 of the bacteria cultured in the microtiter plates was measured for 24 h at 37℃ with vigorous agitation. Data shown are the mean values ± standard deviation (SD) of three independent cultures. UT: untreated, H2O2: H2O2-treated, GSNO: S-nitrosoglutathione-treated.
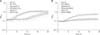
Fig. 2
RpoS-dependent katE transcription in SG. Bacterial cells cultured to log phase in LB media were treated with H2O2 (1 mM) for 30 min. The mRNA levels of katE were measured by quantitative real-time RT-PCR. The values were normalized relative to mRNA levels of a housekeeping gene, gyrB. Data shown are the mean values ± SD of three independent experiments.

Fig. 3
Role of Hmp in nitric oxide (NO) detoxification of SG. (A) NO consumption of the SG strains was recorded by measuring the NO remaining in PBS containing bacteria after the addition of a fast-releasing NO donor, ProliNONOate (2 µM). A NO-sensitive electrode connected to a free radical analyzer was used to measure NO levels as described in the Materials and Methods section. Data are representative of three independent experiments. (B) WT SG cells were cultured in EG media until OD600 = 0.5. Bacterial transcription was then stopped by adding phenol/ethanol solution to the culture before and 1 h after treatment with GSNO (1 mM). Measured values were normalized relative to mRNA levels of the housekeeping gene gyrB. Data shown are mean fold increases ± SD calculated as the ratio of hmp mRNA levels before and after GSNO treatment for three independent experiments.

Fig. 4
SPI-2 gene transcription in SG. mRNA levels of sseJ, ssaB, and sseA were measured using total RNA isolated from SG strains cultured under SPI-2-inducing conditions as described in the Materials and Methods section. Measured values were normalized relative to mRNA levels of the housekeeping gene gyrB. Data shown are mean fold increases ± SD calculated as the ratio of mRNA levels before and after the media change for three independent experiments.
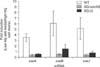
Fig. 5
Attenuation of SGΔ3 in Lohmann layer chickens. The birds were inoculated orally with 1 × 106 (low) or 1 × 108 (high) CFUs of SGΔ3 or 1 × 108 CFUs of WT SG at 2 weeks of age. Bacterial counts in the livers and spleens at 2 weeks post-inoculation are shown as mean values ± SDs of log10 CFU/g for each organ. aIndicates a significant difference (p < 0.0001) between the mutant and WT SG. b,cIndicate a significant difference (p = 0.0008 and p = 0.008) between low and high doses of the SGΔ3 mutant.
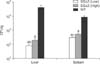
Fig. 6
Protection conferred by SGΔ3 in Lohmann layer chickens. (A) Survival curve for the groups of chickens vaccinated as described in the Materials and Methods section. The chickens were challenged orally at 6 weeks of age with 4 × 108 CFUs of WT SG and observed for 14 days post-challenge (dpc). Bacterial counts in the livers (B) or spleens (C) at 14 dpc are shown as the mean values ± SD of log10 CFU/g for each organ. In the nonvaccinated group, the CFU count was determined for the surviving chickens at 14 dpc. aIndicates a significant difference (p < 0.0001) between SG9R-vaccinated and nonvaccinated chickens. b,cIndicate a significant difference (p = 0.0005 and p = 0.03) between SGΔ3-vaccinated and nonvaccinated chickens. dIndicates a significant difference (p < 0.0001) between SG9R-vaccinated and SGΔ3-vaccinated chickens. e,fIndicate a significant difference (p = 0.0008 and p < 0.01) between SG9R-vaccinated and nonvaccinated chickens. gIndicates a significant difference (p = 0.02) between SGΔ3-vaccinated and nonvaccinated chickens. hIndicates a significant difference (p < 0.001) between SG9R-vaccinated and SGΔ3-vaccinated chickens.

Acknowledgments
This research was financially supported by the Basic Science Research Program through the National Research Foundation of Korea (NRF) funded by the Ministry of Education, Science and Technology (2009-0089873), the Chungcheong Institute for Regional Program Evaluation under the Ministry of Trade, Industry and Energy (MOTIE), the Agricultural Biotechnology Development Program through the Ministry of Agriculture, Food and Rural Affairs (114057-3), and the Korea Institute for Advancement of Technology (KIAT) through the Research and Development for Regional Industry.
References
1. Bang IS, Frye JG, McClelland M, Velayudhan J, Fang FC. Alternative sigma factor interactions in Salmonella: σE and σH promote antioxidant defenses by enhancing σS levels. Mol Microbiol. 2005; 56:811–823.


2. Bang IS, Liu L, Vazquez-Torres A, Crouch ML, Stamler JS, Fang FC. Maintenance of nitric oxide and redox homeostasis by the Salmonella flavohemoglobin Hmp. J Biol Chem. 2006; 281:28039–28047.


3. Barrow PA, Freitas Neto OC. Pullorum disease and fowl typhoid-new thoughts on old diseases: a review. Avian Pathol. 2011; 40:1–13.


4. Bouzoubaa K, Nagaraja KV, Kabbaj FZ, Newman JA, Pomeroy BS. Feasibility of using proteins from Salmonella gallinarum vs. 9R live vaccine for the prevention of fowl typhoid in chickens. Avian Dis. 1989; 33:385–391.


5. Boyen F, Pasmans F, Van Immerseel F, Morgan E, Botteldoorn N, Heyndrickx M, Volf J, Favoreel H, Hernalsteens JP, Ducatelle R, Haesebrouck F. A limited role for SsrA/B in persistent Salmonella Typhimurium infections in pigs. Vet Microbiol. 2008; 128:364–373.


6. Coynault C, Robbe-Saule V, Norel F. Virulence and vaccine potential of Salmonella typhimurium mutants deficient in the expression of the RpoS (σS) regulon. Mol Microbiol. 1996; 22:149–160.


7. Datsenko KA, Wanner BL. One-step inactivation of chromosomal genes in Escherichia coli K-12 using PCR products. Proc Natl Acad Sci U S A. 2000; 97:6640–6645.


8. Deiwick J, Nikolaus T, Erdogan S, Hensel M. Environmental regulation of Salmonella pathogenicity island 2 gene expression. Mol Microbiol. 1999; 31:1759–1773.


9. Fang FC, Libby SJ, Buchmeier NA, Loewen PC, Switala J, Harwood J, Guiney DG. The alternative σ factor KatF (RpoS) regulates Salmonella virulence. Proc Natl Acad Sci U S A. 1992; 89:11978–11982.
10. Foley SL, Johnson TJ, Ricke SC, Nayak R, Danzeisen J. Salmonella pathogenicity and host adaptation in chicken-associated serovars. Microbiol Mol Biol Rev. 2013; 77:582–607.


11. Forrester MT, Foster MW. Protection from nitrosative stress: a central role for microbial flavohemoglobin. Free Radic Biol Med. 2012; 52:1620–1633.


12. Hart TW. Some observations concerning the S-nitroso and S-phenylsulphonyl derivatives of L-cysteine and glutathione. Tetrahedron Lett. 1985; 26:2013–2016.


13. Jeong JH, Song M, Park SI, Cho KO, Rhee JH, Choy HE. Salmonella enterica serovar Gallinarum requires ppGpp for internalization and survival in animal cells. J Bacteriol. 2008; 190:6340–6350.


14. Jones MA, Wigley P, Page KL, Hulme SD, Barrow PA. Salmonella enterica serovar Gallinarum requires the Salmonella pathogenicity island 2 type III secretion system but not the Salmonella pathogenicity island 1 type III secretion system for virulence in chickens. Infect Immun. 2001; 69:5471–5476.


15. Kazmierczak MJ, Wiedmann M, Boor KJ. Alternative sigma factors and their roles in bacterial virulence. Microbiol Mol Biol Rev. 2005; 69:527–543.


16. Kwon HJ, Cho SH. Pathogenicity of SG 9R, a rough vaccine strain against fowl typhoid. Vaccine. 2011; 29:1311–1318.


17. Löber S, Jäckel D, Kaiser N, Hensel M. Regulation of Salmonella pathogenicity island 2 genes by independent environmental signals. Int J Med Microbiol. 2006; 296:435–447.


18. Morgan E, Campbell JD, Rowe SC, Bispham J, Stevens MP, Bowen AJ, Barrow PA, Maskell DJ, Wallis TS. Identification of host-specific colonization factors of Salmonella enterica serovar Typhimurium. Mol Microbiol. 2004; 54:994–1010.


19. Pasmans F, Van Immerseel F, Heyndrickx M, Martel A, Godard C, Wildemauwe C, Ducatelle R, Haesebrouck F. Host adaptation of pigeon isolates of Salmonella enterica subsp. enterica serovar Typhimurium variant Copenhagen phage type 99 is associated with enhanced macrophage cytotoxicity. Infect Immun. 2003; 71:6068–6074.


20. Robbe-Saule V, Algorta G, Rouilhac I, Norel F. Characterization of the RpoS status of clinical isolates of Salmonella enterica. Appl Environ Microbiol. 2003; 69:4352–4358.


21. Seo YS, Lee SH, Shin EK, Kim SJ, Jung R, Hahn TW. Pulsed-field gel electrophoresis genotyping of Salmonella gallinarum and comparison with random amplified polymorphic DNA. Vet Microbiol. 2006; 115:349–357.


22. Silva EN, Snoeyenbos GH, Weinack OM, Smyser CF. Studies on the use of 9R strain of Salmonella gallinarum as a vaccine in chickens. Avian Dis. 1981; 25:38–52.


23. Smith HW. The immunity to Salmonella gallinarum infection in chickens produced by live cultures of members of the Salmonella genus. J Hyg (Lond). 1956; 54:433–439.

