Abstract
Various somatic cell nuclear transfer (SCNT) techniques for mammalian species have been developed to adjust species-specific procedures to oocyte-associated differences among species. Species-specific SCNT protocols may result in different expression levels of developmentally important genes that may affect embryonic development and pregnancy. In the present study, porcine oocytes were treated with demecolcine that facilitated enucleation with protruding genetic material. Enucleation and donor cell injection were performed either simultaneously with a single pipette (simplified one-step SCNT; SONT) or separately with different pipettes (conventional two-step SCNT; CTNT) as the control procedure. After blastocysts from both groups were cultured in vitro, the expression levels of developmentally important genes (OCT4, NANOG, EOMES, CDX2, GLUT-1, PolyA, and HSP70) were analyzed by real-time quantitative polymerase chain reaction. Both the developmental rate according to blastocyst stage as well as the expression levels CDX2, EOMES, and HSP70 were elevated with SONT compared to CTNT. The genes with elevated expression are known to influence trophectoderm formation and heat stress-induced arrest. These results showed that our SONT technique improved the development of SCNT porcine embryos, and increased the expression of genes that are important for placental formation and stress-induced arrest.
Although somatic cell nuclear transfer (SCNT) has been used for various mammalian species, the efficiency of animal cloning is still very low [1,2,3]. In general, SCNT-generated embryos suffer from incomplete reprogramming with epigenetic modifications [4,5,6,7]. Additionally, embryos produced by SCNT or in vitro fertilization show different gene expression patterns when compared to their in vivo counterparts [8,9,10,11]. These effects are thought to result in abnormal development of the SCNT embryos during the peri- and post-implantation stages [10]. Several previous works have attempted to improve SCNT protocols by optimizing the culture medium composition, controlling activation conditions, and improving micromanipulation techniques [4,12,13,14,15,16,17,18,19,20]. Various SCNT protocols have yielded different levels of embryonic development and different gene expression patterns in cloned blastocysts [9,11,13,21,22,23].
The UV exposure of oocytes used to confirm the enucleation process is known to decrease the in vitro developmental potential of SCNT porcine embryos [17]. A novel UV exposure-free enucleation method using demecolcine, which induces oocytes to develop visible extrusions containing chromosomes with polar bodies into the surface, has been introduced for porcine SCNT programs [3,22]. Direct nuclear injection and cell fusion methods have been generally used for introducing a somatic cell nucleus into an enucleated oocyte [9,17,21,24]. Although both techniques result in similar developmental competence in vitro, the cell fusion method is more commonly performed for porcine species. However, the cell fusion method using electric fusion devices requires more complex and longer steps than the direct injection protocol [9,16]. On the other hand, direct injection has been developed into a one-step procedure for which the enucleation and injection steps are performed simultaneously with the same pipette. This 'one-step SCNT' method has been successfully adapted for rat cloning and has improved embryonic development for rhesus monkey cloning [5,23,25].
Several marker genes that are important for embryonic development are associated with pluripotency (OCT4 and NANOG), trophectoderm formation (EOMES and CDX2), stress (HSP70), metabolism (GLUT-1), and translation (PolyA). OCT4 and NANOG are expressed in the inner cell mass of blastocysts [26,27]. CDX2 and EOMES are expressed during formation of the blastocyst trophectoderm, which represents the first step in embryo differentiation [28]. PolyA polymerase plays a role in RNA processing [29]. The length of an mRNA poly(A) tail is crucial for stabilization of the 3' end of the mRNA transcript. Thus, the expression of PolyA is important for mammalian embryonic development [29,30]. Expression of GLUT-1 that encodes the metabolic protein GLUT-1 is significantly decreased in morulae produced in vitro compared to those generated in vivo [31]. The heat shock protein (HSP) family of molecular chaperones is well known for its role in stress adaptation, and assisting in the folding of newly synthesized proteins and their transcripts [32]. HSP70 expression is an indicator of heat shock, and the absence of HSP expression indicates the maladjustment of embryos to environmental change such as heat stress.
In the present study, we assessed the development of porcine embryos produced via SCNT using two cloning method: simplified one-step SCNT (SONT) with demecolcine and conventional two-step SCNT (CTNT). For this, the expression patterns of several marker genes important for embryonic development were analyzed.
All buffer solutions and culture media were obtained from Life technologies (USA). Other inorganic and organic compounds were obtained from Sigma-Aldrich (USA) unless otherwise specified.
Ovaries were collected from 5- to 6-month-old prepubertal gilts (100 ± 10 kg body weight) from a local slaughterhouse, placed in saline at 30~35℃, and transported to the laboratory within 2 h. After washing with saline three times, cumulus-oocyte complexes (COCs) were recovered by aspiration of 2- to 5-mm follicles using an 18-gauge hypodermic needle attached to a 5 mL disposable syringe (Korea Vaccine, Korea). After washing three times in IVM medium, COCs that were enclosed by more than three layers of compact cumulus cells and an evenly granulated ooplasm were selected for in vitro maturation. The 200~250 selected COCs per were cultured in four-well culture dishes (Nunc, Denmark) containing 500 µL of IVM medium under warmed and gas-equilibrated mineral oil for 44 to 46 h at 38.5℃ and 5% CO2. The IVM medium for oocytes was composed of tissue culture medium 199 with Earle's salts and L-glutamine (TCM199; Life technologies) supplemented with 26.2 mM NaHCO3, 3.05 mM glucose, 0.91 mM sodium pyruvate, 0.57 mM L-cysteine, 10 ng/mL epidermal growth factor (EGF), 1 µg/mL insulin, 10 IU/mL equine chorionic gonadotropin (eCG) and human chorionic gonadotropin (hCG), and 0.1% (w/v) polyvinyl alcohol (PVA) [3].
Fibroblasts were recovered from one porcine conceptus, which was assumed to be between embryonic days 28 to 39 in age, collected from a local slaughterhouse. The head, extremities, and internal organs were removed, and the remaining tissues were cut into small pieces (less than 1mm cube). Cells were dispersed by incubation for 20 min at 38.5℃ with 0.25% (v/v) trypsin solution and cultured in Dulbecco's minimum essential medium supplemented with 10% (v/v) fetal bovine serum for 4 day at 38.5℃ in incubator. For each passage, cells were cultured until confluent, disaggregated by incubation in a 0.05% (v/v) trypsin solution for 3 min at 38.5℃, and dispersed into three new dishes. The cells were maintained in culture for at least eight passages. For long-term storage, cells were collected after trypsinization, frozen in freezing medium (DMEM with 20% FBS and 10% DMSO), and stored in liquid nitrogen.
Oocytes with protruding chromosomes (protruding rate, approx. 70%; data not shown) were enucleated with a 12 µm beveled pipette (inner diameter, 10 to 12 µm, Humagen Pipets; Origo, Denmark) in HEPES-buffered TCM199 (hTCM199) supplemented with 7.5 µg/mL cytochalasin B (CB), 0.05 M sucrose, and 0.4 µg/mL demecolcine. The enucleated oocytes (cytoplasts) were washed extensively in hTCM199 and placed in fresh hTCM199 for nuclear injection. The fetal fibroblasts used as donor cells were transferred to a 10% (w/v) polyvinylpyrrolidone-supplemented hTCM199 microdrop (PVP drop, 6 µL) and gently mixed at room temperature. A microdrop of hTCM199 (injection drop) with 15~20 cytoplasts was placed adjacent to the PVP drop, and the drops were covered with light mineral oil to prevent evaporation. Individual fibroblasts in the PVP drops were aspirated into a beveled injection pipette (inner diameter, 10 to 12 µm, Humagen pipets; Origo). The cytoplasmic membrane of the fibroblasts was ruptured by repetitive pipetting. An injection pipette containing the ruptured fetal fibroblasts was moved to the injection drop, and a ruptured fibroblast was injected into an ooplast through the cytoplasmic membrane (Fig. 1A).
Three to four PVP-treated donor cells were aspirated into a 12-µm beveled pipette after cytoplasmic membrane rupture. The demecolcine-treated oocytes with protruding chromosomes were enucleated with the pipette containing the donor cell, and the aspirated chromosomes from the metaphase II plate were expelled from the pipette. A donor karyoplast was injected into the cytoplasm through the hole that had been made during enucleation (Figs. 1B and 2).
After incubation for 60 to 90 min in culture medium for stabilization, electrical activation of the reconstructed oocytes was performed at room temperature using a CF-150/B electro-cell fusion system (BLS, Hungary). The chamber contained two stainless steel electrodes that were 1.0 mm apart and was filled with with activation buffer; 0.26 M mannitol solution supplemented with 0.1 mM MgSO4, 0.05 M CaCl2, and 0.01% (w/v) PVA. The oocytes were activated with a 1.6 kV/cm DC pulse for 40 µseconds in activation buffer. To prevent pseudo-polar body extrusion, the activated oocytes were treated for 5 h at 38.5℃ in North Carolina State University-23 medium (NCSU-23) supplemented with 5 µg/mL CB.
The activated oocytes were cultured in in embryo culture medium NCSU-23 medium supplemented with both 1% (v/v) essential and non-essential amino acids, 4 mg/mL fatty acid-free Fraction V bovine serum albumin (BSA), and 200 µM kinetin. The CB-treated oocytes were washed nine times with embryo culture medium and cultured in 20 µL drops (10 to 15 oocytes per drop) of culture medium for 7 days at 38.5℃ and 5% CO2.
Blastocysts were incubated in 500 µL of washing medium with Hoechst 33342 (1 µg/mL) for 15 min at RT. After staining, the whole blastocysts were mounted in glycerol on a glass slide (Paul Marienfeld, Germany) and flattened under a coverslip (Paul Marienfeld). Digital photographs were obtained using an inverted microscope (Carl Zeiss, Germany) capable of UV illumination and equipped with excitation filters (460 nm for fluorescence, Fig. 3B). The cells in the images were counted.
mRNA was recovered from five to 10 porcine blastocysts on day 7 using an oligo (dT)25 nucleotide attached to magnetic beads (Dynabeads mRNA Purification Kit; Invitrogen) according to the manufacturer's instructions. Briefly, the embryos were resuspended in 100 µL lysis/binding buffer [100 mM Tris-HCl (pH 7.5), 500 mM LiCl, 10 mM EDTA (pH 8.0), 1% Li dodecylsulfate [LiDS], and 5 mM Dithiothreitol] and vortexed at room temperature for 5 min to facilitate embryo lysis and RNA release. An oligo (dT)25 magnetic bead suspension (50 µL) was added to the samples and incubated with shaking at room temperature for 5 min. The hybridized mRNA-oligo (dT) magnetic beads were washed twice with washing buffer A [10 mM Tris-HCl (pH 7.5), 0.15 M LiCl, 1 mM EDTA, and 1% LiDS] and then once with washing buffer B [10 mM Tris-HCl (pH 7.5), 0.15 M LiCl, and 1 mM EDTA]. Finally, the mRNA samples were eluted in 10 µ Diethyl Pyrocarbonate (DEPC)-treated double-distilled water. For first-strand cDNA synthesis, reverse transcription was performed for 1 h at 37℃ in a final reaction volume of 30 µL. The reaction contained purified mRNA, 6 µL of 5× reaction buffer, 1 µL of dNTPs (1.25 mM each), 1 µL of a random hexamer (50 ng/µL), and 1 µL of M-MuLV reverse transcriptase (200 U/µL; Promega, USA).
cDNA produced from the blastocyst mRNA was analyzed using RT-PCR. The expression levels of genes associated with pluripotency (OCT4 and NANOG), trophectroderm formation (CDX2 and EOMES), metabolism (GLUT-1), translation (PolyA), and heat stress (HSP70) were evaluated (Table 1). For optimal quantification, primers were designed using Primer Express software (Applied Biosystems, USA). RT-PCR was performed using an ABI PRISM 7500 system and SYBR Green PCR Master Mix (Applied Biosystems). All points of the standard curve and all samples were run in triplicate as technical replicates. Standard curves were generated using a verified DNA template for porcine GAPDH.
For each run, 1 µL of cDNA was used as template in a reaction mixture containing 5 µL of double-distilled water, 2 µL of each forward and reverse primer (20 pmol/mL), and 10 µL of SYBR Green PCR Master Mix. The following amplification protocol was performed: denaturation at 95℃ for 10 min, 40 cycles of amplification and quantification at 94℃ for 15 seconds and 60℃ for 1 min with a single fluorescence measurement, and a dissociation curve stage (temperature increase of 0.1℃ every 30 sec from 60℃ up to 95℃ with fluorescence measurements taken). Data were analyzed with 7500 System Sequence Detection software (Applied Biosystems). All samples had the same starting quantities of all candidate reference genes based on the standard curves generated for these genes.
Experiments were repeated three to seven times, and the model effect for each treatment was analyzed with a general linear modeling procedure. When a significant model effect was found, treatment effects for each parameter were compared by the least squares method using the Statistical Analysis System (SAS, USA). Data for embryo development and the number of blastocyst cells are expressed as the mean ± standard error of the mean (SEM). Gene expression levels are expressed as the mean ± standard deviation (SD).
The developmental rate of blastocysts produced by SONT was significantly higher than that of blastocysts generated via CTNT (14.6% vs. 10.7%, p < 0.05; Table 2). No significant difference in cleavage rate was observed. The average number of cells in the blastocysts did not differ between the two groups (31.0 ± 3.7 for SONT vs. 32.1 ± 4.0 for CTNT; Fig. 3A).
The expression levels of CDX2 and EOMES (key genes for trophectoderm formation) along with HSP70 (a heat stress-associated gene) were all significantly increased in the SONT blastocysts (p < 0.05). In the SONT group, the levels of CDX2, EOMES, and HSP70 expression were 2.7-, 2.2-, and 3-fold higher, respectively, than those found in the CTNT control. However, the expression patterns of GLUT-1 (metabolism), PolyA (translation), OCT4 (pluripotency), and NANOG (pluripotency) did not differ significantly between the two experimental groups (Fig. 4).
Various SCNT protocols have been studied to develop suitable species-specific methods since oocytes from every mammalian species have different characteristics and gene expression patterns during embryonic development [7]. However, embryos produced by SCNT have a low rate of preimplantation development [1,2,7]. This is thought to cause of implantation failure and fetal defects.
Due to a high lipid granule content, porcine oocytes appeared very dark in color [33,34,35]. During the enucleation step of SCNT, fluorescence microscopy with UV exposure often facilitates the removal of the oocyte nucleus stained with Hoechst 33342 fluorescent dye. Although this staining allows accurate visualization of the metaphase II chromosomes [17], UV exposure may affect early embryonic development [3,17,18,19,22]. Adding demecolcine to the oocytes is a suggested alternative method for enucleation without nuclear staining or UV exposure [3,22]. After treatment with demecolcine, oocytes with visible extrusions comprised of genetic material on the surface can undergo the enucleation steps without harmful exposure to fluorescent dye or UV radiation. This modified cloning method is also referred to as hand-made cloning [18,36].
Rat cloning is successful only when enucleation is performed immediately after injection [25]. A similar technique was also applied to rhesus monkey SCNT, resulting in a two-fold higher blastocyst development rate than that associated with general SCNT [23]. The technique described in the present study was based on enucleation with demecolcine and a simplified one-step method previously used for rat and rhesus monkey cloning, and was modified so that it was more suitable for porcine SCNT. After demecolcine treatment in the SONT group, the protruding chromosomes along with the first polar body were aspirated into the pipette and removed through the zona pellucida. After releasing the aspirated chromosomes and first polar body from the pipette, the donor karyoplast was re-aspirated into the same pipette, introduced into the enucleated oocyte through the same hole in the zona pellucida, and inserted into the cytoplasm. This SONT protocol has the advantage of reducing oocyte damage because less cytoplasm is removed as a result of the demecolcine-aided enucleation. Furthermore, the same hole in the zona pellucida is used for both enucleation and injection without having to re-reposition the oocyte. This technique shortens the duration of micromanipulation. Ensuring that the appropriate volume of cytoplasm remains in the oocyte during SONT is likely to be helpful for reprogramming the donor cell since the oocyte cytoplasm contains factors that affect not only the breakdown of the nuclear envelope but also premature chromosome condensation [5]. Combined with the shortened micromanipulation time, the more complete reprogramming afforded by SONT seems to facilitate the development of SONT-derived porcine embryos.
To determine the developmental potential of the SONT embryos at the molecular level, we measured the expression of several genes including OCT4, NANOG, CDX2, EOMES, HSP70, GLUT-1, and PolyA using RT-PCR. The present study showed that the SONT protocol did not affect embryonic pluripotency since the levels of OCT4 and NANOG expression did not differ between the two experimental groups. Additionally, SONT did not affect the expression of key translation (PolyA) and metabolism (GLUT-1) genes. In contrast, the expression of CDX2 and EOMES, which are related to trophectoderm formation [28], was significantly higher in the SONT blastocysts than the CTNT controls.
Cloned embryos often fail to achieve full-term development because of defective trophectodermal development [37]. EOMES-deficient embryos do not exhibit trophoblast cell outgrowth and die in the uterus when transferred to surrogate pigs [38]. Murine blastocysts with decreased EOMES expression also fail to implant [39]. The overexpression of CDX2 and EOMES in embryonic stem cells induces trophectodermal differentiation [40]. Since CDX2 and EOMES are crucial for trophectoderm formation, expression of these factors is believed to improve implantation and the development of cloned embryos. The increased expression of key trophectoderm-related genes resulting from SONT may support the implantation of SCNT-derived porcine embryos. In addition, HSP70 expression was up-regulated in the SONT porcine embryos compared to the CTNT controls in the present study. A lack of HSP expression in the embryos indicates an inability to accommodate environmental changes such as heat stress, suggesting that SONT embryos are more resistant to these types of stress than CTNT embryos.
In conclusion, results of the present study showed that SONT improves the in vitro development of SCNT-derived porcine embryos. Expression of HSP70 (a gene that helps alleviate heat stress) as well as CDX2 and EOMES (two factors that influence trophectodermal formation) was up-regulated. These data suggested that our SONT technique is more suitable for the production of SCNT-derived porcine embryos with simpler, faster steps compared to conventional SCNT methods. Transfer of a SONT-derived embryo to a surrogate pig will be studied in the future.
Figures and Tables
Fig. 1
Schematic diagram illustrating the SONT (B) and CTNT (A) protocols. The black arrowheads indicate the protruding chromosomes (oval) and first polar body (round). The black arrows indicate the nuclear donor fibroblasts (filled gray circle). SCNT: somatic cell nuclear transfer.
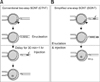
Fig. 2
Stereomicroscopic image of the SONT procedure. The yellow circle (A~C) indicates the oocyte chromosomes and adjacent first polar body. The blue circle (A~D) indicates the nuclear donor cell. Just after aspirating (B) and releasing (C) the oocyte chromosomes and polar body using a micropipette containing the nuclear donor fibroblast, the same pipette was used to immediately introduce the donor cell into the enucleated oocyte. Scale bars = 100 µm.
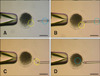
Fig. 3
Comparison of total cell numbers in blastocysts produced by SONT or CTNT. (A) Mean number of total cells in SONT and CTNT blastocysts. (B) Hoechst 33342 staining of the blastocysts (left: CTNT, right: SONT). Scale bar = 100 µm.
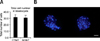
Fig. 4
Expression of genes related to pluripotency, trophoblast formation, metabolism, resistance to heat stress, and translation measured by real-time quantitative polymerase chain reaction. The expression of CDX2 and EOMES (two genes essential for trophectoderm formation) and HSP70 (a heat stress-associated gene) in SONT blastocysts was significantly up-regulated compared to expression levels in the control group (values in CTNT = 1; p < 0.05). Data are expressed as the mean ± SD (n = 3).
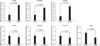
Acknowledgments
This study was supported by a National Research Foundation of Korea Grant funded by the Korean Government (NRF-2006-2004042, and No. 2013070465 through the Oromaxillofacial Dysfunction Research Center for the Elderly at Seoul National University) and the Technology Development Program for Agriculture and Forestry, Ministry of Agriculture, Food and Rural Affairs (MAFRA; 111160-04), Korea.
References
1. Fujimura T, Kurome M, Murakami H, Takahagi Y, Matsunami K, Shimanuki S, Suzuki K, Miyagawa S, Shirakura R, Shigehisa T, Nagashima H. Cloning of the transgenic pigs expressing human decay accelerating factor and N-acetylglucosaminyltransferase III. Cloning Stem Cells. 2004; 6:294–301.


2. Prather RS, Sims MM, First NL. Nuclear transplantation in early pig embryos. Biol Reprod. 1989; 41:414–418.
3. Won C, Park SK, Cho SG, Min BM, Roh S. Kinetin enhances in vitro development of parthenogenetic and nuclear transfer porcine embryos. Mol Reprod Dev. 2008; 75:1701–1709.


4. Li X, Kato Y, Tsuji Y, Tsunoda Y. The effects of trichostatin A on mRNA expression of chromatin structure-, DNA methylation-, and development-related genes in cloned mouse blastocysts. Cloning Stem Cells. 2008; 10:133–142.


5. Mitalipov SM, Zhou Q, Byrne JA, Ji WZ, Norgren RB, Wolf DP. Reprogramming following somatic cell nuclear transfer in primates is dependent upon nuclear remodeling. Hum Reprod. 2007; 22:2232–2242.


6. Morgan HD, Santos F, Green K, Dean W, Reik W. Epigenetic reprogramming in mammals. Hum Mol Genet. 2005; 14:R47–R58.


7. Whitworth KM, Prather RS. Somatic cell nuclear transfer efficiency: how can it be improved through nuclear remodeling and reprogramming? Mol Reprod Dev. 2010; 77:1001–1015.


8. Isom SC, Lai L, Prather RS, Rucker EB 3rd. Heat shock of porcine zygotes immediately after oocyte activation increases viability. Mol Reprod Dev. 2009; 76:548–554.


9. Kawano K, Kato Y, Tsunoda Y. Comparison of in vitro development of porcine nuclear-transferred oocytes receiving fetal somatic cells by injection and fusion methods. Cloning Stem Cells. 2004; 6:67–72.


10. McElroy SL, Kim JH, Kim S, Jeong YW, Lee EG, Park SM, Hossein MS, Koo OJ, Abul Hashem MD, Jang G, Kang SK, Lee BC, Hwang WS. Effects of culture conditions and nuclear transfer protocols on blastocyst formation and mRNA expression in pre-implantation porcine embryos. Theriogenology. 2008; 69:416–425.


11. Wrenzycki C, Wells D, Herrmann D, Miller A, Oliver J, Tervit R, Niemann H. Nuclear transfer protocol affects messenger RNA expression patterns in cloned bovine blastocysts. Biol Reprod. 2001; 65:309–317.


12. Cervera RP, Silvestre MA, Martí N, García-Mengual E, Moreno R, Stojkovic M. Effects of different oocyte activation procedures on development and gene expression of porcine pre-implantation embryos. Reprod Domest Anim. 2010; 45:e12–e20.


13. Costa-Borges N, Paramio MT, Calderón G, Santaló J, Ibáñez E. Antimitotic treatments for chemically assisted oocyte enucleation in nuclear transfer procedures. Cloning Stem Cells. 2009; 11:153–166.


14. Costa-Borges N, Paramio MT, Santaló J, Ibáñez E. Demecolcine- and nocodazole-induced enucleation in mouse and goat oocytes for the preparation of recipient cytoplasts in somatic cell nuclear transfer procedures. Theriogenology. 2011; 75:527–541.


15. García-Mengual E, Alfonso J, Salvador I, Duque CC, Silvestre MA. Oocyte activation procedures and influence of serum on porcine oocyte maturation and subsequent parthenogenetic and nuclear transfer embryo development. Zygote. 2008; 16:279–284.


16. Kurome M, Fujimura T, Murakami H, Takahagi Y, Wako N, Ochiai T, Miyazaki K, Nagashima H. Comparison of electro-fusion and intracytoplasmic nuclear injection methods in pig cloning. Cloning Stem Cells. 2003; 5:367–378.


17. Lee JW, Wu SC, Tian XC, Barber M, Hoagland T, Riesen J, Lee KH, Tu CF, Cheng WTK, Yang X. Production of cloned pigs by whole-cell intracytoplasmic microinjection. Biol Reprod. 2003; 69:995–1001.
18. Li J, Villemoes K, Zhang Y, Du Y, Kragh PM, Purup S, Xue Q, Pedersen AM, Jørgensen AL, Jakobsen JE, Bolund L, Yang H, Vajta G. Efficiency of two enucleation methods connected to handmade cloning to produce transgenic porcine embryos. Reprod Domest Anim. 2009; 44:122–127.


19. Roh S, Choi HY, Park SK, Won C, Kim BW, Kim JH, Kang H, Lee ER, Cho SG. Porcine nuclear transfer using somatic donor cells altered to express male germ cell function. Reprod Fertil Dev. 2009; 21:882–891.


20. Yamanaka K, Sugimura S, Wakai T, Kawahara M, Sato E. Difference in sensitivity to culture condition between in vitro fertilized and somatic cell nuclear transfer embryos in pigs. J Reprod Dev. 2009; 55:299–304.


21. Galli C, Lagutina I, Vassiliev I, Duchi R, Lazzari G. Comparison of microinjection (piezo-electric) and cell fusion for nuclear transfer success with different cell types in cattle. Cloning Stem Cells. 2002; 4:189–196.


22. Yin XJ, Tani T, Yonemura I, Kawakami M, Miyamoto K, Hasegawa R, Kato Y, Tsunoda Y. Production of cloned pigs from adult somatic cells by chemically assisted removal of maternal chromosomes. Biol Reprod. 2002; 67:442–446.


23. Zhou Q, Yang SH, Ding CH, He XC, Xie YH, Hildebrandt TB, Mitalipov SM, Tang XH, Wolf DP, Ji WZ. A comparative approach to somatic cell nuclear transfer in the rhesus monkey. Hum Reprod. 2006; 21:2564–2571.


24. Betthauser J, Forsberg E, Augenstein M, Childs L, Eilertsen K, Enos J, Forsythe T, Golueke P, Jurgella G, Koppang R, Lesmeister T, Mallon K, Mell G, Misica P, Pace M, Pfister-Genskow M, Strelchenko N, Voelker G, Watt S, Thompson S, Bishop M. Production of cloned pigs from in vitro systems. Nat Biotechnol. 2000; 18:1055–1059.


25. Zhou Q, Renard JP, Le Friec G, Brochard V, Beaujean N, Cherifi Y, Fraichard A, Cozzi J. Generation of fertile cloned rats by regulating oocyte activation. Science. 2003; 302:1179.


26. Kirchhof N, Carnwath JW, Lemme E, Anastassiadis K, Schöler H, Niemann H. Expression pattern of Oct-4 in preimplantation embryos of different species. Biol Reprod. 2000; 63:1698–1705.


27. Niwa H, Miyazaki J, Smith AG. Quantitative expression of Oct-3/4 defines differentiation, dedifferentiation or self-renewal of ES cells. Nat Genet. 2000; 24:372–376.


28. Strumpf D, Mao CA, Yamanaka Y, Ralston A, Chawengsaksophak K, Beck F, Rossant J. Cdx2 is required for correct cell fate specification and differentiation of trophectoderm in the mouse blastocyst. Development. 2005; 132:2093–2102.


29. Brevini TAL, Lonergan P, Cillo F, Francisci C, Favetta LA, Fair T, Gandolfi F. Evolution of mRNA polyadenylation between oocyte maturation and first embryonic cleavage in cattle and its relation with developmental competence. Mol Reprod Dev. 2002; 63:510–517.


30. Wormington M. Poly(A) and translation: development control. Curr Opin Cell Biol. 1993; 5:950–954.


31. Niemann H, Wrenzycki C. Alterations of expression of developmentally important genes in preimplantation bovine embryos by in vitro culture conditions: implications for subsequent development. Theriogenology. 2000; 53:21–34.


33. Cran DG. The distribution of organelles in mammalian oocytes following centrifugation prior to injection of foreign DNA. Gamete Res. 1987; 18:67–76.


34. McEvoy TG, Coull GD, Broadbent PJ, Hutchinson JSM, Speake BK. Fatty acid composition of lipids in immature cattle, pig and sheep oocytes with intact zona pellucida. J Reprod Fertil. 2000; 118:163–170.


35. Park SK, Won C, Choi YJ, Kang H, Roh S. The leading blastomere of the 2-cell stage parthenogenetic porcine embryo contributes to the abembryonic part first. J Vet Med Sci. 2009; 71:569–576.


36. Vajta G, Maddox-Hyttel P, Skou CT, Tecirlioglu RT, Peura TT, Lai L, Murphy CN, Prather RS, Kragh PM, Callesen H. Highly efficient and reliable chemically assisted enucleation method for handmade cloning in cattle. Reprod Fertil Dev. 2005; 17:791–797.


37. Lin J, Shi L, Zhang M, Yang H, Qin Y, Zhang J, Gong D, Zhang X, Li D, Li J. Defects in trophoblast cell lineage account for the impaired in vivo development of cloned embryos generated by somatic nuclear transfer. Cell Stem Cell. 2011; 8:371–375.


38. Arnold SJ, Hofmann UK, Bikoff EK, Robertson EJ. Pivotal roles for eomesodermin during axis formation, epithelium-to-mesenchyme transition and endoderm specification in the mouse. Development. 2008; 135:501–511.

