Abstract
Purpose
This study aimed to compare the dosimetric profiles of electron beams (EB) and X-ray beams (XB) for boosting irradiation in breast cancer patients who underwent breast-conserving surgery and postoperative radiotherapy.
Methods
For 131 breast cancer patients who underwent breast-conserving surgery, we compared plans for EB and XB boost irradiation after whole-breast irradiation. The organs at risk (OAR) included the cardiac chambers, coronary arteries, ipsilateral lung, and skin. The conformity index (CI), inhomogeneity index (IHI), and dose-volume parameters for the planning target volume (PTV), and OAR were calculated. Postradiotherapy chest computed tomography scans were performed to detect radiation pneumonitis.
Results
XB plans showed a significantly better CI and IHI for the PTVs, compared to the EB plans. Regarding OAR sparing, the XB reduced the high-dose volume at the expense of an increased low-dose volume. In 33 patients whose radiation fields included nipples, IHI was higher in the EB plans, whereas the presence of a nipple in the radiation field did not interfere with the XB. EB-treated patients developed more subclinical radiation pneumonitis.
Conclusion
XB plans were superior to EB plans in terms of PTV coverage (homogeneity and conformity) and high-dose volume sparing in OAR when used as boost irradiation after breast-conserving surgery. A disadvantage of the XB plan was an increased low-dose volume in the OAR, but this was offset by the increased electron energy. Consequently, tailored plans with either XB or EB are necessary to adapt to patient anatomic variance and tumor bed geometric properties.
The current standard for the treatment of early breast cancer is breast-conserving surgery, followed by whole-breast irradiation and tumor-bed boost irradiation. The benefit of tumor bed boosting has been supported by randomized studies [1,2], although a standard technique has not been clearly established.
The single-field electron beam (EB) plan has been commonly used to deliver a tumor bed boost with a characteristic sharp dose drop-off beyond the target volume [3]. Tumor bed boosting with an X-ray beam (XB) has also been evaluated, but is not widely used. The purpose of this study was to compare the dosimetric parameters of EB plans to those of XB plans for tumor bed boosting. Additionally, the risk of radiation pneumonitis was compared in the selected boost techniques.
One hundred thirty-one women with breast cancer who underwent breast-conserving surgery and adjuvant radiotherapy (RT) between June 2010 and June 2011 were enrolled. Patient and tumor bed characteristics are presented in Table 1. The median patient age was 49 years (range, 24-79 years). Among 131 patients, 61 patients had left-sided breast cancer and 70 patients had right-sided breast cancer. Most patients had early lesions (Table 1).
Before whole-breast RT and tumor bed boost RT, 2 sessions of computed tomography (CT) scanning were performed for treatment planning. Since the lumpectomy cavity volumes changed significantly over the treatment course, additional CT scans were obtained after completing whole-breast irradiation [4,5]. Intravenous contrast-enhanced images were taken to identify the cardiac chambers and coronary arteries. The surgical scars were marked with radio-opaque wires to help define the tumor bed. CT images were then acquired from the mid-neck to the upper abdomen at a 3-mm slice thickness. The CT data were then transferred to a treatment planning system (Eclipse; Varian Medical Systems Inc., Palo Alto, USA).
The clinical target volume (CTV) included the seroma, surgical clips, and scar. In 106 patients, 4 to 6 surgical clips were placed by surgeons to mark the borders of the lumpectomy cavity wall during surgery. Additionally, the architectural distortion under the lumpectomy scar on the chest CT was carefully considered. The planning target volume (PTV) was delineated with a 1- to 1.5-cm margin from the CTV, excluding the pectoralis muscles, chest wall, and a 5-mm depth beneath the skin.
Following whole-breast tangential RT at a dose of 50.4 Gy in 28 fractions, EB and XB treatment plans for boost irradiation were compared in each case. The EB plan used a single electron field with a central axis perpendicular to the PTV. In order for the PTV to be covered by the 90% isodose line, electron energy of 6, 9, 12, 16, or 20 MeV was chosen according to the depth of the PTV. The XB plan comprised 3 conformal XBs of 6 or 10 MV. The gantry and collimator angles were optimized to encompass the PTV by the 95% isodose line while adequately sparing the contralateral breast. The fields were weighted appropriately to maximize sparing of the lung, cardiac chambers, and coronary arteries. Typically, an en face beam was weighted to approximately 70% of the tangential beam. A boost dose of 10 to 16 Gy in daily 2-Gy fractions was delivered according to each patient's surgical margin status.
The organs at risk (OAR) included 4 cardiac chambers, the left ventricle (LV), right ventricle (RV), left atrium (LA), and right atrium (RA), and 3 coronary arteries, specifically the left anterior descending artery (LAD), left circumflex coronary artery (LCX), and right coronary artery (RCA). The ipsilateral lung and a 5-mm-thick skin layer on the ipsilateral breast were also delineated.
The EB and XB plans for each patient were compared via dose-volume histograms (DVH). Target coverage was assessed by comparing the inhomogeneity indices (IHI) and conformity indices (CI) of the plans. The IHI was defined in terms of D5%-D95% (doses received by 5% and 95% of the volume, respectively). The CI was defined as the ratio between the volume receiving at least 95% of the prescribed dose and the volume of the PTV. OAR sparing was evaluated by comparing the mean and maximum doses (Dmean and Dmax, respectively), as well as the dose-volume parameters. A set of appropriate V-values such as V1Gy, V2Gy, V3Gy, and V8Gy (relative volumes receiving ≥1, ≥2, ≥3, or ≥8 Gy of the prescribed dose, respectively) were also calculated. The data from patients with left-sided breast cancer were analyzed to compare doses to the cardiac chambers and coronary arteries. All plans with doses >10 Gy were normalized to deliver 10 Gy within the 95% isodose line after completing RT for the purpose of dosimetric analysis. After comparing the EB and XB plans, we selected 1 treatment plan to deliver boost irradiation.
Post-RT chest CT scans were compared between the EB-treated and XB-treated groups to compare the risk of radiation pneumonitis. Post-RT chest CT scans were available for 81 patients. The median time to chest CT after RT was 6 weeks (range, 5-24 weeks). Each patient's post-RT CT scan was compared to the scan provided for RT planning purposes. Radiation pneumonitis was defined as radiographic opacities within the irradiated volume, including ground-glass opacities or attenuation and consolidation [6,7].
A paired t-test was used to compare paired-sample parameters with regard to target coverage and OAR sparing. The chi-square test was used to compare the prevalence of radiation pneumonitis in the EB-treated and XB-treated groups. The SPSS software program version 18.0 (SPSS Inc., Chicago, USA) was used for all statistical analyses. Null hypotheses of no difference were rejected if the p-values were <0.05.
The mean PTV for all patients was 20.95 cm3 (range, 4.8-88.7 cm3). As shown in Table 2, the PTV coverage of XB plans was superior to that of EB plans. The XB plans provided superior CI, IHI, V95%, and V107% for the PTVs, compared to the EB plans (p<0.05). Moreover, the EB plans showed larger interpatient variability than the XB plans for all indices (Table 2).
Dose distributions were perturbed by nipples in the EB plans. The dosimetric parameters of 33 patients whose RT fields included nipples were evaluated (Table 3). EB plans showed poor IHI and more hot spots (V107%) when a nipple was located in the RT fields (p<0.05). In contrast, XB plans were not affected by the presence of a nipple in the RT fields in terms of the IHI and CI (Table 3, Figure 1).
Table 4 provides the numerical findings from the DVH analysis of the OAR. In terms of cardiac dose, the XB plans significantly increased the Dmean and V1Gy of the LV, compared to the EB plans. On the other hand, the XB plans reduced the V3Gy of the LV, compared to the EB plans (p<0.05). A similar trend was observed for the dosimetric parameters of the LAD. In other words, the XB plans increased the low-dose volumes to the LV and LAD, compared to the EB plans. The quality of the EB plans fluctuated among the patients and was affected by the target depth. Due to the high levels of electron energy required for target coverage, the EB plans overdosed all OAR. The higher the selected electron energy, the lower the difference in LV and LAD sparing between the EB and XB plans (Figure 2). With 6 and 9 MeV electron energy, EB plans showed superior sparing of the LAD and LV. With 12, 16, and 20 MeV, the LV and LAD doses of the EB and XB plans were similar (Figure 2).
The Dmax and Dmean of the lung were higher in the XB plans than in the EB plans (p<0.05) (Table 4). In contrast, the V3Gy of the lung that represented the high-dose volume was higher in the EB plans than in the XB plans (p<0.05). The V2Gy and V8Gy were compared to assess the dose to the skin (Table 4). The V2Gy increased with the XB plans, while the V8Gy increased with the EB plans (p<0.05).
Among the patients with left-sided breast cancers, 32 were treated with EB plans and 29 were treated with XB plans. The proportions of EB-treated and XB-treated patients were near-equal (47.5% and 52.5%, respectively) in patients with left-sided breast cancers. Also among these patients, EB plans were preferred to XB plans in order to decrease the low-dose volumes to the cardiac chambers, coronary arteries, and lungs, although irregular target shapes or deep-seated targets precluded the use of EB. Among patients with right-sided breast cancers, 50 were treated with XB plans and 20 were treated with EB plans. XB plans were preferred to EB plans because of superior homogeneity and conformity (proportions, 71.4% and 28.6%, respectively) in patients with right-sided breast cancers. The actual treatment plans were decided based on the individual tumor location and patient anatomy. EB plans were preferred when the tumor bed was located in the inner breast to avoid irradiating the contralateral breast, or for cases with left outer lower-quadrant tumors to prevent increased low-dose volumes of the cardiac chambers and coronary arteries. In contrast, XB plans were preferred for large PTVs (i.e., deep-seated tumors, obese patients, large breasts, or positive deep margins) or when tumor bed was located under an irregular contour (i.e., nipple, axillary folds, or inframammary folds).
Post-RT chest CT scans were available for 81 of the 131 patients. Symptomatic radiation pneumonitis was not observed. There were no differences in patient characteristics between those patients who underwent post-RT chest CT scans and those who did not (data not shown). The median interval from RT completion to the acquisition of chest CT scans was 6 weeks (range, 5-24 weeks). EB-treated patients were more frequently diagnosed with subclinical radiation pneumonitis on follow-up chest CT scans (p=0.028) (Table 5). None of the patients developed symptomatic pneumonitis or fibrosis that required steroid treatment.
Traditionally, single-field EB plans have been widely used to provide tumor bed boosting to breast cancer patients because EB offers distinct advantages in terms of dose uniformity in the target volume and reducing doses to deeper tissues [3]. However, the limitations of single-field EB plans have been reported by several investigators [8-10]. Kovacs et al. [8] reported that EB plans were inferior to XB plans in terms of target coverage and conformity. Toscas et al. [9] reported that only superficial tumors could be optimally treated with EB plans. The authors noted that high EB energies were required to optimally cover deep-seated PTVs while simultaneously overdosing the skin, heart, breast, and underlying lung. Alexander et al. [10] also reported that EB plans overdosed the OAR when high electron energy was required.
Heart volume sparing is increasingly important in RT for breast cancer patients. With therapeutic strategy improvements, breast cancer patients often survive long enough for long-term cardiac effects to occur. Radiation-associated heart diseases in breast cancer survivors include a wide spectrum of cardiac diseases such as coronary artery disease, myocardial dysfunction, valvular heart disease, pericardial disease, and electrical conduction abnormalities [11-17]. McGale et al. [13] investigated radiation-related cardiac disease in 35,000 women who were treated with RT for breast cancer in Denmark and Sweden. The mortality incidence ratios of left-sided versus right-sided breast cancers were 1.18 for ischemic heart disease (95% confidence interval, 1.7-1.30); 1.61 (95% confidence interval, 1.06-2.43) for pericarditis, and 1.54 (95% confidence interval, 1.11-1.54) for valvular heart disease. Darby et al. [18] reported that the increase in IHD was proportional to the mean dose to the heart, began within a few years after exposure, and continued for at least 20 years. This study revealed that the rates of major coronary events increased linearly with the mean dose to the heart by 7.4% per Gy (95% confidence interval, 2.9-14.5%), with no apparent threshold. Bouchardy et al. [19] analyzed the effects of inner-quadrant irradiation on cardiovascular mortality. Patients with inner-quadrant tumors had a more than doubled risk of cardiovascular mortality, compared to patients with outer-quadrant tumors. In their study, unlike previous studies, patients with left-sided breast cancers had no excess of cardiovascular morality, compared to patients with right-sided breast cancers. Interestingly, the cardiovascular mortality risk was particularly high during the period with higher boost irradiation, suggesting that the boost contributed to the excess of cardiovascular mortality.
We separately analyzed the irradiated doses to the major coronary arteries and cardiac chambers in both XB and EB boost plans. High-dose volumes to the LAD, LV, LA, and LCX were reduced in the XB plans, compared to the EB plans, at the expense of increasing the low-dose volumes. In the EB plans, low-dose volume sparing decreased as the selected electron energy increased (Figure 2). With higher EB energy, the EB plans increased the doses to the LAD and LV. This result agreed with previous reports that suggested that deep-seated tumors might not be adequately treated with EB plans [9,10].
With EB plans, the high-dose volume to the ipsilateral lung increased while low-dose volume decreased. Early pulmonary inflammatory reactions that involve alveolar cell depletion and inflammatory cell accumulation in the interstitial space occur within 4 to 12 weeks [7,20,21]. Wennberg et al. [22] reported that short-term post-RT lung density changes were associated with the irradiated lung doses. Mah et al. [23] reported dose-response curves for acute lung damage at 6 months after RT. In our study, the proportion of patients with subclinical radiation pneumonitis on CT increased in the EB-treated patients at a median interval of 6 weeks after RT completion. This increase might be related to an increase in the high-dose volume to the lung. However, the relatively short follow-up period and the lack of impact from whole-breast RT made it difficult to determine the reliability of the analysis. Further investigations are needed to evaluate the association between the dose-volume parameters and the risk of radiation pneumonitis.
Collette et al. [2] reported that the risk of late skin damage was related to high radiation doses to the skin. In the present study, the high-dose volume to the skin increased with EB plans, compared to XB plans (Table 4). With the megavoltage XB that is presently in use, the surface dose was much smaller than the maximal dose (skin-sparing effect). When compared to XB, the skin-sparing effect with clinical EB was only modest or nonexistent [3]. Reduced high-dose volumes to the skin might reduce the risk of long-term skin effects.
For target coverage, significant differences were found that favored the XB plans. XB plans showed better homogeneity and conformity when compared to the EB plans. Particularly, the presence of a nipple in the RT field negatively affected EB plan target homogeneity and conformity. This might reflect a drawback of EB plans; sharp surface irregularities can produce localized hot and cold spots in the underlying tissue due to scattering. Electrons are predominantly scattered outward by steep projections such as nipples [3]. Single-field EB plans provided poor homogeneity and conformity for target volumes within or around inframammary or axillary folds. To overcome these disadvantages, a bolus material could be used to flatten out an irregular surface, but would result in the loss of the skin-sparing advantage. In this study, we did not place boluses to even out irregular contours.
Tumor bed boost irradiation can be delivered by performing interstitial brachytherapy, either intraoperatively or postoperatively. Local control rates, disease-free survival rates, and cosmesis achieved by EB and brachytherapy when used for delivery to the tumor bed have been compared in several studies, with no significant differences [24-27]. Although the clinical outcomes achieved with either EB or interstitial brachytherapy boost were comparable, considerations of convenience, radiation safety, and cost favored EB radiotherapy. Modern RT techniques such as intensity modulated RT and volumetric modulated arc therapy can also be applied to deliver boost irradiation. Several dosimetric studies [9,10,28] have demonstrated improvements in dose homogeneity with these techniques. However, concerns about higher leakage radiation and higher monitor units that could potentially increase the risk of secondary malignancy, a lack of convincing evidence for better clinical outcomes, and the increased costs reduce the likelihood that these modern techniques will be used for boost irradiation.
The present study aimed to investigate the dosimetric advantages and drawbacks of EB and XB plans. Several practical considerations for tumor bed boosting were identified. First, the tumor bed location should be taken into account. For tumor beds located in the inner breast, EB plans were preferred over XB plans because the contralateral breast was spared. Second, the tumor bed depth should be taken into account. High-energy EB was required to cover the PTV in cases with deep tumor beds. However, higher EB energy resulted in overdoses to the underlying OAR (LA and LV), due to the reduced physical advantage (sharp dose drop-off beyond the target volume). Furthermore, irregular surfaces and contours should also be taken into account. EB was inferior to XB in the presence of a nipple in the RT field or if there was a sudden change in the PTV depth.
XB plans permitted more optimal tumor bed coverage than did EB plans. For OAR sparing, the high-dose volumes increased with EB plans, while the low-dose volumes increased with XB plans. However, the low-dose volume sparing effect of EB was offset by electron energy increases. EB plans were superior for boosting superficial tumor beds, whereas XB plans were superior when the PTV depth was large or the body contour was irregular. A tailored plan with either EB or XB is mandatory to adapt to patient anatomic variances and geometric properties of tumor beds.
Figures and Tables
Figure 1
Isodose distribution in axial planes for one example case in which a nipple perturbed electron beam distribution. (A) Electron beam. (B) X-ray beam. Color line means a 100%, 90%, 80%, 30%, and 10% of the prescribed dose isoline. Red line means planning target volume.
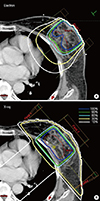
Figure 2
The average dose-volume histograms for left ventricle (column 1) and left anterior descending artery (column 2) in left-sided breast cancer according to the minimum electron energy for covering clinical target volume. (A) 6 MeV. (B) 9 MeV. (C) 12 MeV. (D) 16 MeV.
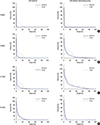
References
1. Romestaing P, Lehingue Y, Carrie C, Coquard R, Montbarbon X, Ardiet JM, et al. Role of a 10-Gy boost in the conservative treatment of early breast cancer: results of a randomized clinical trial in Lyon, France. J Clin Oncol. 1997; 15:963–968.


2. Collette S, Collette L, Budiharto T, Horiot JC, Poortmans PM, Struikmans H, et al. Predictors of the risk of fibrosis at 10 years after breast conserving therapy for early breast cancer: a study based on the EORTC Trial 22881-10882 'boost versus no boost'. Eur J Cancer. 2008; 44:2587–2599.


3. Khan FM. The Physics of Radiation Therapy. 4th ed. Philadelphia: Lippincott Williams & Wilkins;2009. p. 264–310.
4. Kim LH, DeCesare S, Vicini F, Yan D. Effect of lumpectomy cavity volume change on the clinical target volume for accelerated partial breast irradiation: a deformable registration study. Int J Radiat Oncol Biol Phys. 2010; 78:1121–1126.


5. Hurkmans C, Admiraal M, van der Sangen M, Dijkmans I. Significance of breast boost volume changes during radiotherapy in relation to current clinical interobserver variations. Radiother Oncol. 2009; 90:60–65.


6. Libshitz HI, Shuman LS. Radiation-induced pulmonary change: CT findings. J Comput Assist Tomogr. 1984; 8:15–19.
7. Choi YW, Munden RF, Erasmus JJ, Park KJ, Chung WK, Jeon SC, et al. Effects of radiation therapy on the lung: radiologic appearances and differential diagnosis. Radiographics. 2004; 24:985–997.


8. Kovacs A, Hadjiev J, Lakosi F, Glavak C, Antal G, Bogner P, et al. Comparison of photon with electron boost in treatment of early stage breast cancer. Pathol Oncol Res. 2008; 14:193–197.


9. Toscas JI, Linero D, Rubio I, Hidalgo A, Arnalte R, Escudé L, et al. Boosting the tumor bed from deep-seated tumors in early-stage breast cancer: a planning study between electron, photon, and proton beams. Radiother Oncol. 2010; 96:192–198.


10. Alexander A, Soisson E, Hijal T, Sarfehnia A, Seuntjens J. Comparison of modulated electron radiotherapy to conventional electron boost irradiation and volumetric modulated photon arc therapy for treatment of tumour bed boost in breast cancer. Radiother Oncol. 2011; 100:253–258.


11. Seddon B, Cook A, Gothard L, Salmon E, Latus K, Underwood SR, et al. Detection of defects in myocardial perfusion imaging in patients with early breast cancer treated with radiotherapy. Radiother Oncol. 2002; 64:53–63.


12. Harris EE, Correa C, Hwang WT, Liao J, Litt HI, Ferrari VA, et al. Late cardiac mortality and morbidity in early-stage breast cancer patients after breast-conservation treatment. J Clin Oncol. 2006; 24:4100–4106.


13. McGale P, Darby SC, Hall P, Adolfsson J, Bengtsson NO, Bennet AM, et al. Incidence of heart disease in 35,000 women treated with radiotherapy for breast cancer in Denmark and Sweden. Radiother Oncol. 2011; 100:167–175.


14. Rutqvist LE, Lax I, Fornander T, Johansson H. Cardiovascular mortality in a randomized trial of adjuvant radiation therapy versus surgery alone in primary breast cancer. Int J Radiat Oncol Biol Phys. 1992; 22:887–896.


15. Darby SC, McGale P, Taylor CW, Peto R. Long-term mortality from heart disease and lung cancer after radiotherapy for early breast cancer: prospective cohort study of about 300,000 women in US SEER cancer registries. Lancet Oncol. 2005; 6:557–565.


16. Marks LB, Yu X, Prosnitz RG, Zhou SM, Hardenbergh PH, Blazing M, et al. The incidence and functional consequences of RT-associated cardiac perfusion defects. Int J Radiat Oncol Biol Phys. 2005; 63:214–223.


17. Erven K, Jurcut R, Weltens C, Giusca S, Ector J, Wildiers H, et al. Acute radiation effects on cardiac function detected by strain rate imaging in breast cancer patients. Int J Radiat Oncol Biol Phys. 2011; 79:1444–1451.


18. Darby SC, Ewertz M, McGale P, Bennet AM, Blom-Goldman U, Brønnum D, et al. Risk of ischemic heart disease in women after radiotherapy for breast cancer. N Engl J Med. 2013; 368:987–998.


19. Bouchardy C, Rapiti E, Usel M, Majno SB, Vlastos G, Benhamou S, et al. Excess of cardiovascular mortality among node-negative breast cancer patients irradiated for inner-quadrant tumors. Ann Oncol. 2010; 21:459–465.


20. Rodrigues G, Lock M, D'Souza D, Yu E, Van Dyk J. Prediction of radiation pneumonitis by dose-volume histogram parameters in lung cancer: a systematic review. Radiother Oncol. 2004; 71:127–138.


21. Tsoutsou PG, Koukourakis MI. Radiation pneumonitis and fibrosis: mechanisms underlying its pathogenesis and implications for future research. Int J Radiat Oncol Biol Phys. 2006; 66:1281–1293.


22. Wennberg B, Gagliardi G, Sundbom L, Svane G, Lind P. Early response of lung in breast cancer irradiation: radiologic density changes measured by CT and symptomatic radiation pneumonitis. Int J Radiat Oncol Biol Phys. 2002; 52:1196–1206.


23. Mah K, Keane TJ, Van Dyk J, Braban LE, Poon PY, Hao Y. Quantitative effect of combined chemotherapy and fractionated radiotherapy on the incidence of radiation-induced lung damage: a prospective clinical study. Int J Radiat Oncol Biol Phys. 1994; 28:563–574.


24. Bartelink H, Horiot JC, Poortmans P, Struikmans H, Van den Bogaert W, Barillot I, et al. Recurrence rates after treatment of breast cancer with standard radiotherapy with or without additional radiation. N Engl J Med. 2001; 345:1378–1387.


25. Perez CA, Taylor ME, Halverson K, Garcia D, Kuske RR, Lockett MA. Brachytherapy or electron beam boost in conservation therapy of carcinoma of the breast: a nonrandomized comparison. Int J Radiat Oncol Biol Phys. 1996; 34:995–1007.


26. Mansfield CM, Komarnicky LT, Schwartz GF, Rosenberg AL, Krishnan L, Jewell WR, et al. Ten-year results in 1070 patients with stages I and II breast cancer treated by conservative surgery and radiation therapy. Cancer. 1995; 75:2328–2336.

