Abstract
Purpose
Reduced brain glucose metabolism and basal forebrain cholinergic neuron degeneration are common features of Alzheimer's disease and have been correlated with memory function. Although regions representing glucose hypometabolism in patients with Alzheimer's disease are targets of cholinergic basal forebrain neurons, the interaction between cholinergic denervation and glucose hypometabolism is still unclear. The aim of the present study was to evaluate glucose metabolism changes caused by cholinergic deficits.
Materials and Methods
We lesioned basal forebrain cholinergic neurons in rats using 192 immunoglobulin G-saporin. After 3 weeks, lesioned animals underwent water maze testing or were analyzed by 18F-2-fluoro-2-deoxyglucose positron emission tomography.
Results
During water maze probe testing, performance of the lesioned group decreased with respect to time spent in the target quadrant and platform zone. Cingulate cortex glucose metabolism in the lesioned group decreased, compared with the normal group. Additionally, acetylcholinesterase activity and glutamate decarboxylase 65/67 expression declined in the cingulate cortex.
Positron emission tomography (PET) is widely used to evaluate metabolic changes in neurodegenerative disorders.1 PET with 18F-2-fluoro-2-deoxyglucose ([F-18]FDG) as a tracer can confirm neuronal function by demonstrating glucose consumption. Reduced glucose metabolism in specific areas is a common feature of Alzheimer's disease (AD), with reductions in both the hippocampal and cortical regions being demonstrated by [F-18]FDG-PET imaging.23 Basal forebrain cholinergic neuronal degeneration is also a common feature of AD. An association between cholinergic deficits and memory dysfunction has been reported in many clinical studies and animal experiments.45 For example, rats with experimentally lesioned cholinergic neurons show substantial spatial learning and memory impairments.67
Although brain regions representing glucose hypometabolism in AD are target sites of basal forebrain cholinergic neurons, the interaction between cholinergic denervation and glucose hypometabolism is still unclear. Some studies have suggested that even though there is a reduction in glucose metabolism due to cholinergic damage, recovery without cholinergic recovery occurs after 3 weeks to 3 months.8910 However, another study reported reduced glucose metabolism for 4.5 months after cholinergic damage.11
We have previously shown that selective basal forebrain cholinergic neuronal damage by the immunotoxin 192 immunoglobulin G-saporin causes spatial memory impairment, which is related to hippocampal cholinergic dysfunction, as well as damage to the frontal cortex.12 The present study aimed to evaluate glucose metabolism changes caused by basal forebrain cholinergic neuronal damage and confirm the region of dysfunction.
All animal experiments were conducted according to the Guide for the Care and Use of Laboratory Animals of the National Research Council (USA). Rats were housed in plastic cages with food and tap water available ad libitum. A 12-h dark/light cycle was maintained in a temperature and humidity controlled room. All behavioral training and testing occurred during the light phase. Twenty-four male Sprague-Dawley rats weighing 200-250 g were randomly assigned to one of two experimental groups before surgery. The normal group (n=12) underwent no surgical procedure. The lesion group (n=12) received an intraventricular administration of 192 IgG-saporin.
All rats in the lesion group were anesthetized with a mixture of ketamine (75 mg/kg), acepromazine (0.75 mg/kg), and rompun (4 mg/kg), and secured in a stereotaxic frame. After a scalp incision, two holes were drilled in the skull at the stereotaxic coordinates of 0.8 mm posterior and ±1.2 mm lateral from the bregma. Rats were injected bilaterally with 8 µL of 192 IgG-saporin (0.63 µg/µL, Chemicon, Temecula, CA, USA) 3.4 mm below the cortical surface. The solutions were delivered at 1 µL/min. The syringe was left in place for 5 min after injection.
Three weeks after surgery, seven rats from each group were trained in the Morris water maze as previously described.12 Briefly, the testing consisted of training trials for 5 days (4 trials/day) with a platform in a fixed position and a 1 day probe trial without a platform. Rats not reaching the platform within 60 s were led to the platform by the experimenter and allowed to remain there for 10 s. Forty-eight hours after the last training trial, rats underwent the probe trial, lasting 60 s, during which the platform was removed from the pool. Swim paths were recorded using a video tracking system above the center of the pool.
Three weeks after surgery, five rats from each group were deprived of food for 12-15 h to enhance [F-18]FDG uptake in the brain. Each animal was warmed and adapted to a sound-attenuated booth (maintained at 30℃) with 60 dB of white noise for at least 30 min before [F-18]FDG injection. [F-18] FDG (500 mCi/100 g body weight) was injected through the tail vein without anesthesia. After [F-18]FDG uptake (40 min), imaging was performed using a Focus 120 MicroPET system (Concorde Microsystems, Knoxville, TN, USA). During the PET scans (40 min), animals were maintained under isoflurane inhalation anesthesia (2% in 100% oxygen; IsoFlo; Abbott Laboratories, Quebec, Canada).
After behavioral testing, 10 rats from each group were perfused with cold 4% paraformaldehyde. Their brains were removed, post-fixed, and placed in 30% sucrose for 3 days. The brains were sectioned at 30 µm using a freezing microtome and stored in cryoprotectant solution [-20℃; 0.1 M phosphate buffer (pH 7.2), 30% sucrose, 1% polyvinylpyrrolidone, and 30% ethylene glycol]. Anatomical landmarks were used to localize the medial septum (MS) and cingulate cortex (CC).13 To detect choline acetyltransferase (ChAT)-immunopositive cells in the MS, brain sections were immunohistochemically processed using polyclonal antibodies against ChAT (Chemicon, Temecula, CA, USA). To detect GABAergic cells in the CC, brain sections were processed using glutamate decarboxylase (GAD) 65/67 polyclonal antibodies (Millipore, Temecula, CA, USA).
To acquire protein for the acetylcholinesterase (AChE) assay, two rats from each group were anesthetized with a mixture of ketamine, acepromazine, and rompun. The brains were quickly removed and the CC dissected to yield a sample in a 1 mm coronal brain slice. The samples were homogenized in lysis buffer (Intron, Seongnam, Korea) and centrifuged for 15 min at 13000 rpm. Protein in the supernatant was measured using a bicinchoninic acid protein assay reagent kit (Pierce, Rockford, IL, USA). Protein samples were stored at -20℃ and the enzymatic activity of AChE determined using the method of Ellman, et al.14 with some modifications. Briefly, triplicate samples (20 µL) were added to the reaction mixture [0.2 mM dithiobisnitrobenzoic acid (Sigma, St. Louis, MO, USA), 0.56 mM acetylthiocholine iodide (Sigma), 10 µM tetraisoprpylpyrophosphoramide (Sigma), and 39 mM phosphate buffer, pH 7.2] at 37℃. After 30 min, the optical density (OD) was measured at 405 nm.
Water maze probe test results were expressed as a percentage of the normal group. An independent t test was used to analyze for significant differences between the two groups. A p value <0.05 was considered statistically significant. All statistical analyses were performed using PASW software (version 18; SPSS Inc., Chicago, IL, USA). For statistical analysis, brain regions in the [F-18]FDG microPET images were manually extracted. Individual images were normalized using the [F-18] FDG rat brain template. To make the [F-18]FDG rat brain templates, normal rat brain images (n=12) were co-registered to the respective images and re-sliced with trilinear interpolation (0.4×0.4×0.4 mm3) using SPM5 (http://www.fil.ion.ucl.ac.uk/spm). Values from individual images were averaged to make the [F-18]FDG rat brain template. It was then normalized to an MRI template for accurate anatomical information in stereotaxic space.15 Voxel-based statistical analyses were carried out between the normal and lesion groups using a t test.
The statistical threshold was set at p<0.05 (family-wise error correction) with an extent threshold of 100 contiguous voxels. T value maps of results were overlaid on transverse views of the MRI template to define voxels showing significant changes. For correlation analyses among the brain areas with significant metabolic changes, the average glucose metabolism of all voxels in each activated or deactivated brain region was calculated at the Paxino's position of each region.
Intraventricular 192 IgG-saporin injections produced ChAT-immunopositive neuronal denervation in the MS. ChAT-immunopositive neurons in normal rats were evenly distributed in the MS, with the cell body structure and dendrites completely intact (Fig. 1A). In contrast, the lesion group showed a remarkable decrease in the number of ChAT-immunopositive neurons and severe damage to the cell bodies and dendrites (Fig. 1B).
On the first training trial day, the latencies of normal and lesion group rats to reach the platform were 34.9 and 38.1 s, respectively, which was not significantly different (Fig. 2A). Both groups showed similar latencies (12 s) on the last training trial day, suggesting that latency to reach the platform declined progressively across training days and both groups progressively learned the hidden platform location. During the probe test (Fig. 2B), the lesion group showed no difference from the normal group in motor-related behaviors, as evidenced by similar swim distances and speeds. These findings suggest cholinergic lesions do not affect motor function. However, the amount of time spent by the lesion group in the target quadrant and platform zone decreased to 60% (p<0.05) and 36% (p<0.05) of normal group values, respectively. These differences were statistically significant. Moreover, the number of platform crossings decreased to 38% of the normal group, though this difference was not statistically significant.
Fig. 3 shows changes in glucose metabolism in coronal (Fig. 3A-F), horizontal (Fig. 3G), and sagittal (Fig. 3H) sections in rats. Coronal sections indicating glucose hypometabolism were located 0.6 mm to -1.4 mm from bregma (Fig. 3A-F). A significant decrease (p<0.05) in glucose metabolism was found in the bilateral cingulate and motor cortices of the lesion group. There were no significant differences in glucose metabolism of other regions, including the hippocampus and basal forebrain.
In the normal group, AChE activity in the CC was measured as an OD of 0.56. In contrast, the lesion group had an OD value of 0.54. This difference was significant (p<0.05) (Fig. 4), suggesting cholinergic neuron damage in the basal forebrain by 192 IgG-saporin reduced CC cholinergic activity.
In this study we showed that animals with sufficient basal forebrain cholinergic neuronal damage to cause spatial memory impairment exhibit a reduction in CC glucose metabolism and a reduction of both the cholinergic and GABAergic systems.
Basal forebrain cholinergic projections to the CC are verified by histologic studies. Electrolytic lesions of the MS and diagonal band region reduce diffuse AChE staining in the CC.16 A study using a fluorescent AChE tracer showed that pathways to the CC are derived from all subdivisions of the diagonal band and nucleus basalis of Meynert (NBM).17 Animal tests examining the relationship between basal forebrain cholinergic neuron deficits and cerebral glucose metabolism have been conducted. In a comparative study on uni- and bilateral NBM damage, unilateral damage did not cause a permanent reduction in cortical metabolism, whereas bilateral damage caused a persistent suppression of cortical glucose metabolism.18 A study of cholinergic neuronal damage via bilateral intraventricular injection of 192 IgG-saporin reported sustained hippocampal and cortical hypometabolism for 4.5 months.11 In this study, damaged cholinergic neurons showed a significant reduction in CC glucose metabolism that was maintained for 3 weeks. However, in a study of left NBM damage in baboons, even though significant metabolic depression of the entire ipsilateral CC was observed 4 days after lesioning, glucose metabolism slowly recovered to almost normal limits within 6-13 weeks.8 Hippocampal glucose metabolism was profoundly suppressed on the third week, but returned to control values after 3 months.9 After either uni- or bilateral NBM lesions with ibotenic acid, glucose metabolism decreased on the third day and recovered between 3 weeks to 3 months.101920 From studies of reported hypometabolism recovery, it appears possible to spared cholinergic function in intact areas by damaging only the NBM or MS. Alternatively, intraventricular injection of 192 IgG-saporin only damages cholinergic cells expressing nerve growth factor receptor p75, likely damaging most cholinergic projections from the basal forebrain to the cortex or hippocampus. Thus, differences in hypometabolism recovery may originate from different methods of cholinergic lesion.
It is well documented that the hippocampus plays an essential role in the formation of memory.2122 However, extrahippocampal structures, especially neocortical regions, have been investigated for crucial roles in long-term memory.2324 Animal studies show that a reduction in anterior CC (ACC) is associated with remote memory. Expression of c-fos, which is related to neuronal activity, increases after remote memory water maze testing, but not with recent memory testing.25 In addition, pharmacological inactivation of the ACC impairs retrieval of 15- and 29-day memories,26 and electrophysiological activity of ACC neurons is increased while testing long-term memory.27 Furthermore, long-term fornix/hypothalamus deep-brain stimulation has been shown to enhance memory in patients with AD through memory circuit activation and altered ACC glucose metabolism.28 Together with the above studies, spatial memory impairment concurrent with ACC dysfunction supports an important role for the ACC in long-term memory.
Memory impairment following a basal forebrain cholinergic lesion appears related to glutamatergic, GABAergic, and cholinergic system dysfunction. The CC has receptors for acetylcholine, GABA, and glutamate.2930 The fact that neurotransmitter systems in the CC are related to memory processing has been reported in a pharmacological study.31 Additionally, the initial increase and subsequent reduction of GABA during cognitive testing has been reported.32 Glucose metabolism, measured by PET, appears closely associated with glutamate-driven activation of cells.33 Furthermore, animal research has examined the effects of basal forebrain cholinergic damage in the glutamatergic system. Some animal studies have reported decreases in cortical and hippocampal glutamatergic transmissions after NBM lesions,34 whereas others have reported different responses based on the type and location of glutamatergic receptors.3536
No significant differences in hippocampal glucose metabolism we observed in this study. In our previous study, we evaluated 192 IgG-saporin effects on the expression of activityregulated cytoskeleton associated protein (Arc) and GAD. We found they were significantly decreased in the frontal cortex, but not in the hippocampus.12 The effects of basal forebrain cholinergic deficits caused by 192 IgG-saporin appear to be greater in the frontal cortex than the hippocampus. However, hippocampal and cortical hypometabolism is reported 18 weeks after a selective cholinergic lesion.11 Additionally, it is well known that there is a close connection between basal forebrain cholinergic neurons, hippocampal function, and spatial memory.37 Therefore, we cannot exclude hippocampal dysfunction from involvement in memory impairment.
Glucose hypometabolism in the [F-18]FDG-PET results was observed in the CC and motor cortex. However, we could not confirm differences between groups regarding motor-related behaviors (swim distance and speed). In our previous study, we evaluated the effects of 192 IgG-saporin by dose on swim speed and distance. In the 10 µL-injection group, both the speed and distances in water maze testing were slightly decreased compared to the normal group, though the differences were not significant.12 Thus, there may be motor dysfunction which could not evaluate with water maze testing, because we observed motor cortex dysfunction, using [F-18]FDG-PET, following 8-µL injection of 192 IgG-saporin.
This study shows the effects of cholinergic dysfunction on cognitive function (including spatial memory) and CC function. The CC is known for its role in regulation of mood, such as depression.38 We attempted to deduce if deficits in cholinergic levels induced abnormal mood regulation via reduced CC function in patients with AD. AD presents with various behavioral and psychological symptoms besides cognitive dysfunction.39 The cause for these other symptoms may be accounted for by various mechanisms, such as amyloid plaques and intraneuronal neurofibrillary tangles.40 This study shows that mood changes in patients with AD may be the direct result of cholinergic dysfunction. It proposes that cholinergic restoration could treat mood changes in AD. Unfortunately, we did not perform experiments to confirm this hypothesis in this study. Further studies of cholinergic restoration are needed to provide additional evidence for its therapeutic effects on mood change.
In conclusion, we examined spatial memory impairment in an animal model mimicking cholinergic denervation seen in patients with AD. Cholinergic denervation appears to be associated with a decline in the functions of GABAergic, cholinergic, and glutamatergic systems, correlating with glucose hypometabolism in the CC.
Figures and Tables
Fig. 1
Representative images showing effects of the cholinergic lesion. (A) The normal group has numerous ChAT-immunopositive neurons in the MS. (B) The lesion group displays a loss of cholinergic neurons in the MS. Scale bar represents 500 µm. ChAT, choline acetyltransferase; MS, medial septum.
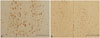
Fig. 2
Cholinergic deficit effects on spatial memory. (A) Latency indicates the time required for the rat to find the escape platform during training trials. All groups showed a similar latency of 10 s on the last day of the training trial, suggesting they remembered the platform location. Data are shown as mean±standard error of the mean. (B) During the probe test, the time spent in the target quadrant (*p<0.05) and in the platform zone (*p<0.05) is significantly different between the lesion and normal groups. Indices are expressed as the percentage of normal group values.
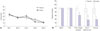
Fig. 3
Changes in glucose metabolism in coronal (A-F), horizontal (G), and sagittal (H) brain sections from rats. Significant declines (p<0.05) in glucose metabolism are seen in the bilateral cingulate and motor cortices of the lesion group.
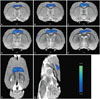
Fig. 4
Cholinergic deficit effects on AChE activity in the CC. AChE activity of the lesion group was significantly decreased compared with the normal group (*p<0.05). AChE activity is expressed as the OD at 405 nm. Values are the mean±standard error of the mean. AChE, acetylcholinesterase; OD, optical density; CC, cingulate cortex.
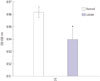
Fig. 5
Representative images showing decreased GAD65/67 positive cells. (A) GAD65/67-immunopositive cells in normal rats are densely distributed in the CC. (B) The lesion group shows a notable decline of GAD65/67-immunopositive cells. Scale bar represents 500 µm. CC, cingulate cortex; GAD, glutamate decarboxylase.
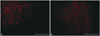
ACKNOWLEDGEMENTS
This work was supported by a grant from the Korea Healthcare Technology R&D Project, Ministry of Health & Welfare, Republic of Korea (A120300, J. W. Chang), and also supported by basic-research fund from Medtronic Korea Co. Ltd. (W. S. Chang).
References
2. Mosconi L, Mistur R, Switalski R, Tsui WH, Glodzik L, Li Y, et al. FDG-PET changes in brain glucose metabolism from normal cognition to pathologically verified Alzheimer's disease. Eur J Nucl Med Mol Imaging. 2009; 36:811–822.


3. Demetriades AK. Functional neuroimaging in Alzheimer's type dementia. J Neurol Sci. 2002; 203-204:247–251.


4. Bierer LM, Haroutunian V, Gabriel S, Knott PJ, Carlin LS, Purohit DP, et al. Neurochemical correlates of dementia severity in Alzheimer's disease: relative importance of the cholinergic deficits. J Neurochem. 1995; 64:749–760.


5. Whitehouse PJ, Price DL, Clark AW, Coyle JT, DeLong MR. Alzheimer disease: evidence for selective loss of cholinergic neurons in the nucleus basalis. Ann Neurol. 1981; 10:122–126.


6. Nilsson OG, Leanza G, Rosenblad C, Lappi DA, Wiley RG, Björklund A. Spatial learning impairments in rats with selective immunolesion of the forebrain cholinergic system. Neuroreport. 1992; 3:1005–1008.


7. Croxson PL, Kyriazis DA, Baxter MG. Cholinergic modulation of a specific memory function of prefrontal cortex. Nat Neurosci. 2011; 14:1510–1512.


8. Kiyosawa M, Baron JC, Hamel E, Pappata S, Duverger D, Riche D, et al. Time course of effects of unilateral lesions of the nucleus basalis of Meynert on glucose utilization by the cerebral cortex. Positron tomography in baboons. Brain. 1989; 112:435–455.


9. Harrell LE, Davis JN. Cholinergic denervation of the hippocampal formation does not produce long-term changes in glucose metabolism. Exp Neurol. 1984; 85:128–138.


10. Ouchi Y, Fukuyama H, Ogawa M, Yamauchi H, Kimura J, Magata Y, et al. Cholinergic projection from the basal forebrain and cerebral glucose metabolism in rats: a dynamic PET study. J Cereb Blood Flow Metab. 1996; 16:34–41.


11. Browne SE, Lin L, Mattsson A, Georgievska B, Isacson O. Selective antibody-induced cholinergic cell and synapse loss produce sustained hippocampal and cortical hypometabolism with correlated cognitive deficits. Exp Neurol. 2001; 170:36–47.


12. Jeong da U, Chang WS, Hwang YS, Lee D, Chang JW. Decrease of GABAergic markers and arc protein expression in the frontal cortex by intraventricular 192 IgG-saporin. Dement Geriatr Cogn Disord. 2011; 32:70–78.


13. Paxinos G, Watson C. The rat brain in stereotaxic coordinates. 4th ed. San Diego (CA): Academic Press;1998.
14. Ellman GL, Courtney KD, Andres V Jr, Feather-stone RM. A new and rapid colorimetric determination of acetylcholinesterase activity. Biochem Pharmacol. 1961; 7:88–95.


15. Schweinhardt P, Fransson P, Olson L, Spenger C, Andersson JL. A template for spatial normalisation of MR images of the rat brain. J Neurosci Methods. 2003; 129:105–113.


16. Stewart DJ, MacFabe DF, Leung LW. Topographical projection of cholinergic neurons in the basal forebrain to the cingulate cortex in the rat. Brain Res. 1985; 358:404–407.


17. Bigl V, Woolf NJ, Butcher LL. Cholinergic projections from the basal forebrain to frontal, parietal, temporal, occipital, and cingulate cortices: a combined fluorescent tracer and acetylcholinesterase analysis. Brain Res Bull. 1982; 8:727–749.


18. Katsumi Y, Hanakawa T, Fukuyama H, Hayashi T, Nagahama Y, Yamauchi H, et al. The effect of sequential lesioning in the basal forebrain on cerebral cortical glucose metabolism in rats. An animal positron emission tomography study. Brain Res. 1999; 837:75–82.


19. Ogawa M, Iida Y, Nakagawa M, Kuge Y, Kawashima H, Tominaga A, et al. Change of central cholinergic receptors following lesions of nucleus basalis magnocellularis in rats: search for an imaging index suitable for the early detection of Alzheimer's disease. Nucl Med Biol. 2006; 33:249–254.


20. Katsumi Y, Hayashi T, Oyanagi C, Nagahama Y, Yamauchi H, Ono S, et al. Glucose metabolism in the rat frontal cortex recovered without the recovery of choline acetyltransferase activity after lesioning of the nucleus basalis magnocellularis. Neurosci Lett. 2000; 280:9–12.


21. Burgess N, Maguire EA, O'Keefe J. The human hippocampus and spatial and episodic memory. Neuron. 2002; 35:625–641.


22. Nakazawa K, McHugh TJ, Wilson MA, Tonegawa S. NMDA receptors, place cells and hippocampal spatial memory. Nat Rev Neurosci. 2004; 5:361–372.


23. Teng E, Squire LR. Memory for places learned long ago is intact after hippocampal damage. Nature. 1999; 400:675–677.


24. Frankland PW, O'Brien C, Ohno M, Kirkwood A, Silva AJ. Alpha-CaMKII-dependent plasticity in the cortex is required for permanent memory. Nature. 2001; 411:309–313.


25. Teixeira CM, Pomedli SR, Maei HR, Kee N, Frankland PW. Involvement of the anterior cingulate cortex in the expression of remote spatial memory. J Neurosci. 2006; 26:7555–7564.


26. Liu F, Zheng XL, Li BM. The anterior cingulate cortex is involved in retrieval of long-term/long-lasting but not short-term memory for step-through inhibitory avoidance in rats. Neurosci Lett. 2009; 460:175–179.


27. Weible AP, Rowland DC, Monaghan CK, Wolfgang NT, Kentros CG. Neural correlates of long-term object memory in the mouse anterior cingulate cortex. J Neurosci. 2012; 32:5598–5608.


28. Laxton AW, Tang-Wai DF, McAndrews MP, Zumsteg D, Wennberg R, Keren R, et al. A phase I trial of deep brain stimulation of memory circuits in Alzheimer's disease. Ann Neurol. 2010; 68:521–534.


29. Bijak M. Daily changes in the mouse cingulate cortex responsivity to neurotransmitters and depolarizing medium: an extracellular in vitro study. Gen Pharmacol. 1988; 19:107–111.


30. Jones RS, Olpe HR. Multiple changes in the sensitivity of cingulate cortical neurones to putative neurotransmitters in ageing rats: substance P, acetylcholine and noradrenaline. Neurosci Lett. 1984; 50:31–36.


31. Farr SA, Uezu K, Creonte TA, Flood JF, Morley JE. Modulation of memory processing in the cingulate cortex of mice. Pharmacol Biochem Behav. 2000; 65:363–368.


32. Kohl MM, Paulsen O. The roles of GABAB receptors in cortical network activity. Adv Pharmacol. 2010; 58:205–229.


33. Pellerin L, Magistretti PJ. Glutamate uptake into astrocytes stimulates aerobic glycolysis: a mechanism coupling neuronal activity to glucose utilization. Proc Natl Acad Sci U S A. 1994; 91:10625–10629.


34. Reine G, Samuel D, Nieoullon A, Kerkerian-Le Goff L. Effects of lesion of the cholinergic basal forebrain nuclei on the activity of glutamatergic and GABAergic systems in the rat frontal cortex and hippocampus. J Neural Transm Gen Sect. 1992; 87:175–192.


35. Rossner S, Schliebs R, Bigl V. 192IgG-saporin-induced immunotoxic lesions of cholinergic basal forebrain system differentially affect glutamatergic and GABAergic markers in cortical rat brain regions. Brain Res. 1995; 696:165–176.


36. Nicolle MM, Shivers A, Gill TM, Gallagher M. Hippocampal N-methyl-D-aspartate and kainate binding in response to entorhinal cortex aspiration or 192 IgG-saporin lesions of the basal forebrain. Neuroscience. 1997; 77:649–659.


37. Ikonen S, McMahan R, Gallagher M, Eichenbaum H, Tanila H. Cholinergic system regulation of spatial representation by the hippocampus. Hippocampus. 2002; 12:386–397.


38. Alexopoulos GS, Gunning-Dixon FM, Latoussakis V, Kanellopoulos D, Murphy CF. Anterior cingulate dysfunction in geriatric depression. Int J Geriatr Psychiatry. 2008; 23:347–355.

