Abstract
Purpose
Adipose-derived stem cells (ADSCs) are known to be potentially effective in regeneration of damaged tissue. We aimed to assess the effectiveness of intracoronary administration of ADSCs in reducing the infarction area and improving function after acute transmural myocardial infarction (MI) in a porcine model.
Materials and Methods
ADSCs were obtained from each pig's abdominal subcutaneous fat tissue by simple liposuction. After 3 passages of 14-days culture, 2 million ADSCs were injected into the coronary artery 30 min after acute transmural MI. At baseline and 4 weeks after the ADSC injection, 99mTc methoxyisobutylisonitrile-single photon emission computed tomography (MIBI-SPECT) was performed to evaluate the left ventricular volume, left ventricular ejection fraction (LVEF; %), and perfusion defects as well as the myocardial salvage (%) and salvage index. At 4 weeks, each pig was sacrificed, and the heart was extracted and dissected. Gross and microscopic analyses with specific immunohistochemistry staining were then performed.
Results
Analysis showed improvement in the perfusion defect, but not in the LVEF in the ADSC group (n=14), compared with the control group (n=14) (perfusion defect, -13.0±10.0 vs. -2.6±12.0, p=0.019; LVEF, -8.0±15.4 vs. -15.9±14.8, p=0.181). There was a tendency of reducing left ventricular volume in ADSC group. The ADSCs identified by stromal cell-derived factor-1 (SDF-1) staining were well co-localized by von Willebrand factor and Troponin T staining.
Stem cells which were isolated from adipose tissue have been suggested as a new cell source in regenerative medicine. Evidences have shown that treatment with adipose tissue-derived stem cells (ADSCs) improved heart function.1234 The putative mechanisms involved are believed to be the ability of ADSCs to differentiate into vascular endothelial cells with its associated paracrine effects.5678910 Both cultured ADSCs and the stromal vascular fraction (SVF) form of adipose tissue-derived cells (ADCs) were found to effectively improve heart function.3
Although many other resources of stem cells including bone marrow-derived mesenchymal stem cells (BM-MSCs), mesenchymal stem cells, and myoblasts have been evaluated in the area of regenerative medicine within the field of cardiology.5111213 ADSCs have a variety of unique benefits. ADSCs are abundant in the body, relatively easy to harvest without concern of complications, and convenient compared to the BM-MSCs via bone marrow biopsy.14 They are also well cultured and expanded with high potency compared to other stem cell resources.78915 Also, they are multipotent cell sources.11151617 Most of all, they are free of ethical problems.911 Preclinical and clinical studies evaluating the effect of ADSCs in acute myocardial infarction (MI) have recently been performed.1234 However, less information is available about the effect of ADSCs in reducing left ventricle (LV) remodeling and chamber size. Here, we evaluated the efficacy of cultured ADSCs in improving myocardial perfusion and function as well as the effect on the LV remodeling in a large animal acute MI model to explore its potential therapeutic application in humans.
This study protocol was approved by the animal experimental committee of Pusan National University Hospital. A total of 28 pigs were analyzed. Among them, 14 were in the study group (ADSC group), while the other 14 were in the control group. They were followed up for 4 weeks after the induction of MI. The perfusion defect, myocardial salvage (%), and salvage index (calculated from the perfusion defect), left ventricular ejection fraction (LVEF), and left ventricular chamber volume were evaluated using 99mTc methoxyisobutylisonitrile (MIBI) scan at baseline and at the 4-week follow-up examination. Wall thickness of the left ventricle (LV), gross infarct area of the ventricles, capillary density, and immunohistochemistry (IHC) staining were also evaluated after pigs were sacrificed. This study was single-blinded, and the operator was not able to know the group difference.
Adipose tissues were obtained by simple liposuction from the abdominal subcutaneous fat under general inhalation anesthesia isoflurane (1-3%) just 2 weeks before MI induction. Subcutaneous adipose tissues were digested with collagenase I (1 mg/mL) under gentle agitation for 60 min at 37℃. The digested tissues were centrifuged, and the pellets (SVF) were resuspended in Dulbecco's modified Eagle's medium (Invitrogen, Carlsbad, CA, USA)-based media containing 0.2 mmol/L ascorbic acid and 10% fetal bovine serum (FBS) obtained from a bovine spongiform encephalopathy-free herd. The cell fraction was cultured overnight at 37℃/5% CO2 in the above media. After 24 h, the cell medium was changed to a keratinocyte-serum-free medium (Invitrogen)-based medium containing 0.2 mmol/L ascorbic acid, 0.09 mmol/L calcium, 5 ng/mL recombinant epidermal growth factor, and 5% FBS. The cells were subculture-expanded in the same media until passage 3.
In our earlier studies, we examined the characteristics of the ADSCs, including surface marker expression and angiogenic factor secretion.567 FBS contaminants from the cultured ADSCs were completely removed by several washings with phosphate-buffered saline (PBS), and then the albumin concentration was determined using a bovine albumin enzyme-linked imimmunosorbent assay quantification kit to determine if the level was below the detection limit (Bethyl Laboratories, Montgomery, TX, USA).
The Korea Food and Drug Administration permitted the use of the FBS-eliminated ADSCs for this clinical study. Aliquots of the ADSCs were then tested for cell viability and fungal, bacterial, endotoxin, and mycoplasma contamination as required by the Code of Federal Regulations, Title 21 (21 CFR) before further use. The ADSC were prepared at the good manufacturing practice (GMP) laboratory of RNL Bio (Seoul, Korea) under GMP conditions. The in vitro hypoxia experiments were performed with a hypoxic incubator (APM-30D; ASTEC, Fukuoka, Japan) that continuously infuses a calibrated gas mixture (95% N2, 5% CO2). The experiments were performed at oxygen concentrations of 21% and 1%. After all processes, the ADSCs were tagged with CM-dil (Vybrant®, Invitrogen, Carlsbad, CA, USA) fluorescence.
To characterize the phenotypes of the ADCs, a flow cytometry analysis was performed. At least 50000 cells (in 100 µL of PBS, 0.5% bovine serum albumin, and 2 mmol/L ethylenediaminetetraacetic acid) were incubated with fluorescence-labeled monoclonal antibodies against human Flk-1, CD90, CD31, CD34, CD44, and CD45 (BD Biosciences, CA, USA) or the respective isotype control (1/20 dilution; 4℃, 30 min). After washing steps, the labeled cells were analyzed by flow cytometry using a FACSCalibur flow cytometer and CellQuest Pro software (BD Biosciences).
The MI model was made under the general isoflurane inhalation anesthesia (1-3%) and a ketamine intramuscular injection (20 mg/kg). All of the pigs were pre-medicated with 2.5 mg bisoprolol, 100 mg aspirin, and 75 mg clopidogrel daily for 3 days before the ADSC administration. Before the procedure, 10000 units of intravenous heparin were administered. After the procedure, no medication was administered thereafter. The percutaneous approach was used via the right or left femoral artery. Transmural MI was induced with balloon occlusion [angioplasty over-the-wire (OTW) coaxial balloon, 2.5×20 mm] at the mid left anterior descending artery (LAD) after a second diagonal branch for 3 h under electrocardiography (ECG) and hemodynamic monitoring (Fig. 1). Intravenous amiodarone was administered at a dose of 150 mg to prevent lethal arrhythmia during the procedure. Thirty minutes after the balloon deflation, 2×106 of ADSCs were injected via an OTW balloon for 3 min with balloon packing. To prevent slow or no-reflow phenomenon, total dose of ADSCs divided by 3 times was delivered into coronary artery. After a 5-min period to allow for the recovery of coronary flow in the two groups, a final angiograms were performed. After reperfusion period, a dose of 1110 MBq 99mTc-MIBI was injected via a femoral sheath to access the perfusion defect and jeopardize the myocardium with a scintigraphic examination. Except for the administration of ADSCs, all the managements were same for the control group.
After the procedure, the pigs were directly transferred for the 99mTc MIBI-scan evaluation while still under anesthesia. Imaging was performed with each pig in a supine position under ECG and hemodynamic monitoring using a single head nuclear gamma camera (Phillips Vertex; ADAC Laboratories/Philips Medical Systems, Milpitas, CA, USA). The images were acquired with a circular 180° acquisition of 64 projections with an acquisition time of 30 s per image. From the raw data, dedicated software (AutoSPECT+InStill 5.0, Ultra Myocardial Display Version 3.41 and AutoQUANT 5.1; ADAC Laboratories, CA, USA) was used to analyze the LV parameters including LV wall motion and wall thickening. The myocardial perfusion defect (%) was evaluated at baseline and at the follow-up examination, and myocardial salvage was obtained using the following simple calculation: (initial perfusion defect-final perfusion defect)/initial perfusion defect. Myocardial salvage index was defined as the ratio of myocardial salvage (%)/initial perfusion defect (%). LVEF and LV volumes were also analyzed and compared.
To evaluate the gross infarct area and the degree of wall thickness, digital images of every heart slice were documented. Infarct area and wall thickness were demarcated and calculated. The mean of every infarct area on each gross specimen was acquired and presented as total infarct area/total ventricular area (%). The wall-thickness ratio was obtained from the ratio of the infarct area wall thickness to the non-infarct area wall thickness.
The myocardial tissues of the infarct border zone were removed and fixed in neutral buffered 4% (w/v) paraformaldehyde at 4℃ for 24 h prior to being embedded in paraffin and sectioned. Transverse muscular sections (5 µm) were deparaffinized and then stained with hematoxylin and eosin. The slides were examined at ×200 magnification (×20 objective lenses with ×10 digital camera). To investigate the ability of ADSCs to differentiate into endothelial cells and cardiomyocytes, various IHC evaluations were performed: stromal cell-derived factor-1 (SDF-1), and CD44 as a mesenchymal origin surface marker, von Willebrand factor (vWF) and CD31 as an angiogenesis marker, and TnT as a cardiomyocyte regeneration marker.
Initially, a total of 109 female pigs aged 8 weeks were enrolled, but 50% of pigs (n=55) died during the acute phase of MI. Another 24% of pigs (n=26) died during the follow up examination or period. There was no significant difference in the number of dropout pigs between two groups. Finally, 28 pigs (14 in the ADSC group, 14 in the control group) survived after 4 weeks and were analyzed. At the 4-week follow-up examination, ADSC engraftment was noticed in all pigs, evidenced by CM-dil fluorescence tagging under fluorescence microscopy at ×40 magnification (Fig. 2).
The degree of improvement of the perfusion defect at follow-up examination was significantly greater in the ADSC group (-13.0% in the ADSC group vs. -2.6% in the control group, p=0.019) (Table 1, Fig. 3). Sequentially, the degree of myocardial salvage and the myocardial salvage index were numerically higher in the ADSC group, but the difference was not statistically significant [myocardial salvage (%), 31.0±24.0 in the ADSC group vs. 9.8±39.3 in the control group, p=0.097; myocardial salvage index, 0.7±0.7 in the ADSC group vs. 0.4±1.3 in the control group, p=0.345].
LVEF (%) decreased from 43.9% at baseline to 35.9% at the follow-up examination in the ADSC group and from 54.7% to 38.9% in the control group. Although the initial LVEF was significantly lower in the ADSC group, the LVEF at 4 week follow up was similar among two groups. The change in LVEF (%) between the baseline and follow-up was lower in the ADSC group without statistical significance (-8.0±15.4 in the ADSC group vs. -15.9±14.8 in the control group, p=0.181).
A volumetric evaluation showed a tendency of reduced dilatation of LV chamber size in the ADSC group. Both left ventricular end diastolic volume (LVEDV) and left ventricular end systolic volume (LVESV) increased at final examination in both groups, but the change of LV volume was numerically smaller in the ADSC group without significance. [ΔLVEDV (mL), 20.4±20.3 in the ADSC group vs. 42.0±26.0 in the control group, p=0.109; ΔLVESV (mL), 14.3±15.3 in the ADSC group vs. 32.3±27.4 in the control group, p=0.155] (Table 1).
Gross specimens of 24 pigs (12 in the ADSC group and 12 in the control group) were analyzed. The mean wall thickness ratio was not different between the two groups (0.6±0.2 cm in the ADSC group vs. 0.6±0.2 cm in the control group; p=0.375). The infarct area was smaller in the ADSC group with marginal significance (13.9±10.6 cm2 in the ADSC group vs. 22.7±13.2 cm2 in the control group; p=0.085) (Fig. 4).
This study showed that ADSC treatment after acute MI was associated with improved LV perfusion defects and might be associated with improved LV remodeling in a porcine MI model. The major mechanism of this improvement might be the ability of ADSCs to differentiate into vascular endothelial cells and the associated paracrine effect rather than direct myocardial regeneration effect as we affirmed in this study.
Valina, et al.1 earlier evaluated the efficacy of ADSCs in an acute MI model. It was the first study of efficacy and safety of cultured ADSCs in an acute MI model compared with BM-MSCs. Using a 99mTc MIBI scan as well as the LVEF change using an LV angiogram, they showed that cultured ADSCs significantly reduced perfusion defects and improved LVEF at the 4-week follow-up examination compared to that observed at baseline, and also proved that the paracrine effect is the major underlying mechanism with IHC staining. Cultured ADSCs showed efficacy similar to that of BM-MSCs.
Our present study is based on the study of Valina, et al.,1 and our major results support the applicability of the intracoronary injection of ADSCs in an acute MI model. In our study, perfusion defects and myocardial salvage measured by 99mTc methoxyisobutylisonitrile-single photen emission computed tomography (MIBI-SPECT) were significantly improved, but there was no statistical significance in the LVEF change. In addition, in contrast to the study of Valina, et al.,1 our result showed that the LVEF difference was negative in each group and no statistical difference between the two groups. There could be several explanations for this difference. First, because our study is small and low-powered one, inconclusive result might have been rendered. Second, we evaluated the LVEF change using 99mTc MIBI-SPECT, not LV angiography. Evaluation of LV systolic function with scintigraphy might not be as accurate as LV angiography or echocardiography. Third, the animals in our study were not administered any medication after the MI; therefore, our MI model showed worsening LV systolic function, in contrast to improved LV systolic function after acute MI in other studies. Although the pattern of LVEF differed from those of other studies and there were no statistically significant differences, our study showed much lower LVEF deterioration in the ADSC group compared to that in the control group, suggesting that the use of ADSCs might diminish the degree of LV deterioration. Finally, the effect of ADSCs on improvement of LVEF might be small or less. Recent interim analysis of first-in-men study of ADSCs in ST-segment elevation myocardial infarction patients supports such suggestion.4 They showed that ADSCs in freshly isolated form significantly improved the LV perfusion and reduced infarct area, but there was no significant improvement of LVEF measured with various imaging methods. Also, there could be a matter of dosage. Usually up to 10 million cells can be safely delivered into each coronary vessel without significant major micro-infarction or severe embolization. However, in the Valina's previous study comparing the efficacy between BMMSCs and ADSCs, they used 2 millions of stem cells and showed efficacy similar to that of previous studies which evaluated various types of higher dose stem cells. We decided to use lower dose of ADSCs based on the Valina's study. Methodological issue in delivering the stem cells is beyond the scope of this study.
In this study, we measured the LV volume for evaluation of LV remodeling using 99mTc MIBI scan. The amount of LV dilatation that presented with LVEDV and LVESV in the ADSC group was half that of the control group. The tendency toward reduced LVEF deterioration in the ADSC group was due to improved LV dilatation effect, that has beneficial effect on LV remodeling. The effect of ADSCs on LV dilatation improvement was also due to much smaller infarct area in the ADSC group compared to those in the control group.
The mechanisms of ADSCs in cardiac injury are not fully understood, but two major mechanisms seem to be involved: the direct differentiation effect and the paracrine effect. Differentiation into cardiomyocytes was proven in some studies with BMMSC,181920 but not in other studies.21 In our study, however, IHC staining did not reveal a definite marker for cardiomyocytes. Our results are in agreement with those of studies by Silva, et al.22 and Valina, et al.1
Improved cardiac function does not appear to be related to direct differentiation into cardiomyocytes. Instead, direct differentiation into vascular endothelial cells, incorporation into vessels, and neovascularization at the infarct border zone might be the major mechanism for improving cardiac function and remodeling. Our results support this mechanism and are consistent with those of earlier studies. Finally, the paracrine effect also contributes to improved cardiac function. Yang, et al.23 recently evaluated the relative contribution of the paracrine effect and angiogenesis in an animal MI model and revealed that the paracrine effect might be the major mechanism of ADSC function in MI.
Our study has some limitations. First, there is a big difference in baseline LVEF between two groups (almost 10%). That could be explained by technical inexperience, especially early on the study period. Second, we did not evaluate the safety profile of ADSC use. However, many earlier studies concerning the safety of the intracoronary injection of ADSCs revealed that it does not appear to increase the risk of slow flow caused by micro-infarction, lethal arrhythmia, or further MI events, especially when small numbers of cells are delivered.12425 We infused 2 million cells divided by 3 times to minimize the risk of microinfarction by ADSCs. No adverse events including slow- or noreflow phenomenon, confirmed by angiographic evaluation, were noted while injecting ADSCs through the coronary artery. Most adverse events occurred during the transfer of the pigs to the scintigraphy laboratory and consisted of ventricular fibrillation and refractory cardiogenic shock resulting from large anterior wall infarction. Therefore, the intracoronary injection of ADSCs appears to be safe. However, its intrinsic property can cause a problem. That is, facilitated uptake into the infarct myocardium could be impeded by diseased atherosclerotic coronary vasculature which blocks intrinsic cardiomyocyte regenerating characteristics of the ADSCs. In other words, the efficacy of the delivery method, not the safety, is what matters.
The application of cultured human ADSCs in acute clinical settings is also a potential limitation since several days are needed to prepare cultured ADSCs. For this reason, the practical use of allogenic human ADSCs is under investigation. An alternative plan is the use of freshly isolated human adipose tissue-derived cells (fhADCs). Although several studies have presented the efficacy of cultured ADSCs in the protection against MI,12 one study compared the effect of cultured ADSCs and fhADCs as the SVF.3 Both types of cells were administered via intramyocardial injection after the surgical ligation of murine LAD and well engrafted into the infarcted myocardium; the cells not only survived but also improved myocardial function. With these encouraging results, the APOLLO trial, the first human phase I/IIa study evaluating the feasibility and safety of fhADCs, was implemented and the results are pending. An interim analysis showed that the intracoronary injection of fhADCs was feasible and safe, and showed a trend toward improved cardiac function and perfusion defects, and reduced myocardial scarring.4
In conclusion, this study demonstrated that intracoronary administration of cultured ADSCs improved myocardial perfusion defects, reduced LV chamber dilatation and infarct area in a porcine acute MI model. The underlying mechanism might involve the ability of ADSCs to differentiate into vascular endothelial cells with its associated paracrine effect.
Figures and Tables
![]() | Fig. 1Porcine acute myocardial infarction (MI) model. Transmural MI was induced with balloon occlusion [angioplasty over-the-wire (OTW) coaxial balloon, 2.5×20 mm] at the mid left anterior descending artery (LAD) after a second diagonal branch for 3 h under electrocardiography (ECG) and hemodynamic monitoring. |
![]() | Fig. 2Engrafted adipose tissue-derived stem cells tagged with CM-dil in the border zone after 4 weeks (original magnification, ×40). ADSC, adipose tissue-derived stem cell. |
![]() | Fig. 3Nuclear imaging. The degree of improvement of the perfusion defect at follow-up examination was significantly greater in the ADSC group on 99mTc MIBI-scan. ADSC, adipose tissue-derived stem cell; MIBI, methoxyisobutylisonitrile. |
![]() | Fig. 4Comparison of infarct area from gross specimens. Infarct territory was much smaller in the ADSC group. ADSC, adipose tissue-derived stem cell. |
![]() | Fig. 5Identification of phenotype of engrafted adipose tissue-derived stem cells in the infarct border zone by immunohistochemical staining for SDF-1, CD44, vWF, CD31 (original magnification, ×200). Staining with SDF-1, CD44, vWF, and CD31 were more enhanced in the ADSC group compared to the control group. Pattern of the TnT staining was not different between the two groups. ADSC, adipose tissue-derived stem cell; vWF, von Willebrand factor; SDF-1, stromal cell-derived factor-1. |
![]() | Fig. 6Comparison of capillary density after immunohistochemical staining for vWF (original magnification, ×200). Capillary density was higher in the ADSC group than in the control. ADSC, adipose tissue-derived stem cell; vWF, von Willebrand factor. |
Table 1
Comparisons of Parameters from Scintigraphic Study between Two Groups
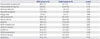
ACKNOWLEDGEMENTS
This work was supported by clinical research grant from Biomedical Research Institute, Pusan National University Hospital (2013).
References
1. Valina C, Pinkernell K, Song YH, Bai X, Sadat S, Campeau RJ, et al. Intracoronary administration of autologous adipose tissue-derived stem cells improves left ventricular function, perfusion, and remodelling after acute myocardial infarction. Eur Heart J. 2007; 28:2667–2677.


2. Cai L, Johnstone BH, Cook TG, Tan J, Fishbein MC, Chen PS, et al. IFATS collection: human adipose tissue-derived stem cells induce angiogenesis and nerve sprouting following myocardial infarction, in conjunction with potent preservation of cardiac function. Stem Cells. 2009; 27:230–237.


3. Bai X, Yan Y, Song YH, Seidensticker M, Rabinovich B, Metzele R, et al. Both cultured and freshly isolated adipose tissue-derived stem cells enhance cardiac function after acute myocardial infarction. Eur Heart J. 2010; 31:489–501.


4. Houtgraaf JH, den Dekker WK, van Dalen BM, Springeling T, de Jong R, van Geuns RJ, et al. First experience in humans using adipose tissue-derived regenerative cells in the treatment of patients with ST-segment elevation myocardial infarction. J Am Coll Cardiol. 2012; 59:539–540.


5. Lee RH, Kim B, Choi I, Kim H, Choi HS, Suh K, et al. Characterization and expression analysis of mesenchymal stem cells from human bone marrow and adipose tissue. Cell Physiol Biochem. 2004; 14:311–324.


6. Moon MH, Kim SY, Kim YJ, Kim SJ, Lee JB, Bae YC, et al. Human adipose tissue-derived mesenchymal stem cells improve postnatal neovascularization in a mouse model of hindlimb ischemia. Cell Physiol Biochem. 2006; 17:279–290.


7. Cho HH, Kim YJ, Kim JT, Song JS, Shin KK, Bae YC, et al. The role of chemokines in proangiogenic action induced by human adipose tissue-derived mesenchymal stem cells in the murine model of hindlimb ischemia. Cell Physiol Biochem. 2009; 24:511–518.


8. Gnecchi M, Zhang Z, Ni A, Dzau VJ. Paracrine mechanisms in adult stem cell signaling and therapy. Circ Res. 2008; 103:1204–1219.


9. Miranville A, Heeschen C, Sengenès C, Curat CA, Busse R, Bouloumié A. Improvement of postnatal neovascularization by human adipose tissue-derived stem cells. Circulation. 2004; 110:349–355.


10. Planat-Benard V, Silvestre JS, Cousin B, André M, Nibbelink M, Tamarat R, et al. Plasticity of human adipose lineage cells toward endothelial cells: physiological and therapeutic perspectives. Circulation. 2004; 109:656–663.


11. Pittenger MF, Mackay AM, Beck SC, Jaiswal RK, Douglas R, Mosca JD, et al. Multilineage potential of adult human mesenchymal stem cells. Science. 1999; 284:143–147.


12. Wang JS, Shum-Tim D, Galipeau J, Chedrawy E, Eliopoulos N, Chiu RC. Marrow stromal cells for cellular cardiomyoplasty: feasibility and potential clinical advantages. J Thorac Cardiovasc Surg. 2000; 120:999–1005.


13. Wakitani S, Saito T, Caplan AI. Myogenic cells derived from rat bone marrow mesenchymal stem cells exposed to 5-azacytidine. Muscle Nerve. 1995; 18:1417–1426.


14. Rehman J, Traktuev D, Li J, Merfeld-Clauss S, Temm-Grove CJ, Bovenkerk JE, et al. Secretion of angiogenic and antiapoptotic factors by human adipose stromal cells. Circulation. 2004; 109:1292–1298.


15. Madonna R, Geng YJ, De Caterina R. Adipose tissue-derived stem cells: characterization and potential for cardiovascular repair. Arterioscler Thromb Vasc Biol. 2009; 29:1723–1729.
16. Zuk PA, Zhu M, Mizuno H, Huang J, Futrell JW, Katz AJ, et al. Multilineage cells from human adipose tissue: implications for cell-based therapies. Tissue Eng. 2001; 7:211–228.


17. Liechty KW, MacKenzie TC, Shaaban AF, Radu A, Moseley AM, Deans R, et al. Human mesenchymal stem cells engraft and demonstrate sitespecific differentiation after in utero transplantation in sheep. Nat Med. 2000; 6:1282–1286.


18. Orlic D, Kajstura J, Chimenti S, Jakoniuk I, Anderson SM, Li B, et al. Bone marrow cells regenerate infarcted myocardium. Nature. 2001; 410:701–705.


19. Kajstura J, Rota M, Whang B, Cascapera S, Hosoda T, Bearzi C, et al. Bone marrow cells differentiate in cardiac cell lineages after infarction independently of cell fusion. Circ Res. 2005; 96:127–137.


20. Dimmeler S, Zeiher AM, Schneider MD. Unchain my heart: the scientific foundations of cardiac repair. J Clin Invest. 2005; 115:572–583.


21. Kuethe F, Richartz BM, Sayer HG, Kasper C, Werner GS, Höffken K, et al. Lack of regeneration of myocardium by autologous intracoronary mononuclear bone marrow cell transplantation in humans with large anterior myocardial infarctions. Int J Cardiol. 2004; 97:123–127.


22. Silva GV, Litovsky S, Assad JA, Sousa AL, Martin BJ, Vela D, et al. Mesenchymal stem cells differentiate into an endothelial phenotype, enhance vascular density, and improve heart function in a canine chronic ischemia model. Circulation. 2005; 111:150–156.


23. Yang D, Wang W, Li L, Peng Y, Chen P, Huang H, et al. The relative contribution of paracine effect versus direct differentiation on adipose-derived stem cell transplantation mediated cardiac repair. PLoS One. 2013; 8:e59020.

