Abstract
Purpose
Guinea pig is one of the most suitable animal models for Mycobacterium tuberculosis (M. tb) infection since it shows similarities to pulmonary infection in humans. Although guinea pig shows hematogenous spread of M. tb infection into the whole body, immunological studies have mainly focused on granulomatous tissues in lungs and spleens. In order to investigate the time-course of disease pathogenesis and immunological profiles in each infected organ, we performed the following approaches with guinea pigs experimentally infected with M. tb over a 22-week post-infection period.
Materials and Methods
We examined body weight changes, M. tb growth curve, cytokine gene expression (IFN-γ and TNF-α), and histopathology in liver, spleen, lungs and lymph nodes of infected guinea pigs.
Results
The body weights of infected guinea pigs did not increase as much as uninfected ones and the number of M. tb bacilli in their organs increased except bronchotracheal lymph node during the experimental period. The gene expression of IFN-γ and TNF-α was induced between 3 and 6 weeks of infection; however, kinetic profiles of cytokine gene expression showed heterogeneity among organs over the study period. Histophathologically granulomatous lesions were developed in all four organs of infected guinea pigs.
Conclusion
Although IFN-γ and TNF-α gene expression profiles showed heterogeneity, the granuloma formation was clearly observed in every organ regardless of whether the number of bacilli increased or decreased. However, this protective immunity was accompanied with severe tissue damage in all four organs, which may lead to the death of guinea pigs.
Tuberculosis (TB) remains as a serious problem worldwide, killing approximately 1.3-2 million people every year worldwide. Based on the World Health Organization report, one-third of the world population are infected with Mycobacterium tuberculosis (M. tb), and 8.7 million new cases of TB occurred in 2011.1-3 For the prevention of TB, bacillus Calmette-Guérin (BCG) has been commonly administered to the people as a vaccine for several decades. However, the efficacy of BCG is highly variable (0-90%)4-7 and the therapeutic drugs currently in use are not effective for the treatment of multi-drug resistant TB or extensively drug-resistant TB.8-11 Therefore, the development of TB vaccines and therapeutic drugs with a high protective efficacy is urgently needed.12-15
In order to investigate the efficacy of vaccines and effectiveness of drugs for TB, we need to understand the pathogenesis of TB and immune evasion by M. tb more comprehensively. Rabbits, guinea pigs and mice are widely used animal models for TB. Although the monkey model is currently an excellent animal model of human TB, this model has substantial limitations in terms of high cost of monkeys and the maintenance of facilities.16,17 Among these animal models, guinea pig is a relevant animal model of human TB since guinea pig is highly susceptible to M. tb and the spectrum of human TB can be biologically and pathologically displayed when infected with M. tb.18-24
The major host defense mechanism for TB consists of cell mediated immune responses (CMI) comprising Th-1 type cytokine response (IFN-γ, TNF-α and IL-12) and granuloma formation at the site of disease. In general, macrophages infected with M. tb are activated by Th-1 type cytokines released by surrounding lymphocytes and they can destroy or inactivate the invading bacilli by producing nitrogen intermediates, lysosomal enzymes and other substances to protect the host from the infection.25-27 In addition, the disease dissemination is contained by the formation of granuloma, which provides the physical barrier for the spreading of bacilli by deposition and fusion of various immune cells.28-30
Guinea pig shows a high sensitivity to M. tb-derived antigens such as tuberculin, and usually develops strong delayed type hypersensitivity (DTH) associated with extensive tissue damage, caseation and necrosis upon M. tb infection. DTH is an another form of CMI, producing IFN-γ, TNF-α and other cytokines. In case of DTH response, however, CMI is induced too robustly so that defense immune response costs the host itself to a great extent.31 Therefore, this inefficient defense mechanism allows the dissemination of TB into the whole body of guinea pigs, usually resulting in deaths.14,19
To understand the mechanism leading to the development of TB and host defense responses in vivo in each infected organ, we investigated two aspects of host responses to M. tb infection in guinea pigs in this study.30,32 First, we studied the kinetics of IFN-γ and TNF-α gene expression in each organ infected with M. tb during 22 weeks post-infection periods since IFN-γ and TNF-α are crucial Th-1 type cytokines controlling the TB infection.33,34 Secondly, we examined the relationship of these cytokine responses with the pathogenicity since the disease progression in TB can be regulated by cytokine production at the site of disease and the cytokine responses can also be affected by the severity of TB.
Hartley guinea pigs (pathogen-free female, outbred) were purchased from the Japan SLC, Inc. (Shizuoka, Japan) and maintained under the barrier conditions in a biohazard animal room at the Yonsei University Medical Research Center. The guinea pigs were 3-4 weeks old at the time of challenge and exposed to M. tb strain H37Rv in an inhalation chamber (Glas-Col. Inc., Terre Haute, IN, USA) to which about 106 colony forming units (CFUs) in 5 mL were aerolized. Animals were sacrificed at 1, 3, 6, 10, 14 and 22 weeks post-infection to obtain each organ, and body weights were measured at every sacrificed time point. Accordingly, the number of animals which were weighed was different at each time point (0 and 1 week: 9, 3 wk: 7, 6 wk: 5, 10 wk: 3, 14 wk: 2 and 22 wk: 1 animal).
Groups of 1-2 randomly selected guinea pigs were killed at 1, 3, 6, 10, 14 and 22 weeks post-infection. One half of the lung, spleen and bronchotracheal lymph nodes (BTLNs) and one-sixth of liver were separately homogenized. Homogenates were serially diluted 10-folds and plated onto Middlebrook 7H11 (Difco Laboratories, Detroit, MI, USA) agar plates, which were incubated at 37℃ for 14-21 days. CFU value was determined from the counts unchanged for three consecutive weeks.
Tissues (lung, spleen, liver and BTLN) were fixed by immersion in 10% neutral buffered formalin. Samples were embedded in paraffin, and cut into sections at 5 µm. Sections were then stained with hematoxylin and eosin and examined by light microscopy.
The primers used in this experiment were as follows: β-actin (590 bp: ATGTGCAAGGCCGGCTTCG and ATGTCACGCACAATTTCC) (genbank accession No: AF508792), IFN-γ (206 bp: GCCAAATCGTCTCCTTCTAC and GAGTTCAATGATCGCTTGGCGCTGGA) (genbank accession No: AY151287, and TNF-α (351 bp: GAGCTCGCAGAGGAG and CACCAGCTGGTTGTCGCTCAGGCC) (genbank accession No: U77036). To quantitatively analyze cytokine gene expression, total RNA was extracted from four tissues (lung, BTLN, spleen and liver) at selected time points post-infection using a RNeasy Mini Kit (Qiagen, Valencia, CA, USA). Total RNA (1 µg) was reverse-transcribed into cDNA using AccuPower RT/PCR PreMix (Bioneer, Seoul, Korea) and the synthesized cDNA (1 µL) was used for PCR amplification of the gene for β-actin, IFN-γ or TNF-α. The resulting PCR-products were separated on an agarose gel, stained with ethidium bromide and quantified by densitometry (G: BOX EF2) (Syngene, Frederick, MD, USA). By calculating the ratio of actual value of target gene to the reference gene, β-actin, the relative expression of IFN-γ or TNF-α was analyzed.
In order to establish slowly progressive primary TB, guinea pigs were infected with low dose of M. tb. Each guinea pig was weighed for various periods of time and compared with age-matched uninfected controls. At the beginning of the experiment, they weighed approximately 300 to 400 grams. As shown in Fig. 1, the body weight of the animals either infected or uninfected increased with time, but the increase was less in infected guinea pigs compared to uninfected control, although the sample sizes were too small to analyze statistically. The uninfected guinea pigs increased progressively in weight with time, gaining approximately 730 g by 22 weeks from the beginning of the experiment, whereas the infected guinea pigs reached only a mean of approximately 620-670 g. These data, together with the observation of abdominal respiration in the animals 1-week post-infection, suggest that M. tb infection affected the growth rate of guinea pigs, resulting in growth restraint in chronic infection.
We examined the kinetics of the mRNA expression of type 1 cytokines, IFN-γ and TNF-α, at each organ at various time points in response to the aerosol infection. Total RNA was extracted from each organ and cDNA was reverse-transcribed. Both IFN-γ and TNF-α specific mRNAs were amplified by RT-PCR. As shown in Fig. 2, the basal level expression of both IFN-γ and TNF-α genes was observed in the organs of uninfected ones (0 week). The expression levels of IFN-γ in lungs, BTLNs, and spleens of infected animals reached peak levels at 3 weeks post-infection and were induced 7-20 folds higher than those in uninfected ones. The level was maintained at different periods in each organ. The IFN-γ expression in the liver was lower than other organs and reached peak levels at 6 weeks post-infection, which was 5.8 folds higher than that in uninfected ones.
The expression of TNF-α mRNA was efficiently induced at around 3 to 6 weeks post-infection in each organ and this was maintained up to 10 weeks in each organ except in the liver. In spleen and liver, the mRNA expression kinetics of TNF-α was similar to that of IFN-γ. The kinetics of IFN-γ and TNF-α gene expression in the spleen was somewhat inversely proportional to the growth curve of M. tb after infection.
M. tb growth in each organ of guinea pig was monitored by plate count at the times indicated. After one week of the aerosol infection, bacilli multiplied rapidly to three logs of the initially inoculated size in the lungs of guinea pig as illustrated in Fig. 3. At this stage, any growth of M. tb was not observed in other organs. However, in three weeks after infection, bacilli were disseminated into spleen, liver and lymph node, and the bacilli growth in each organ was logarithmic over the first three weeks of the experiment, as shown in other reports.19 The maximum growth was observed in the lung and lymph node at around week 3. At the same time, mRNA expression of key cytokines, IFN-γ and TNF-α, for cell-mediated immune responses was induced and then subsequently bacterial growth reached the stationary phase, achieving about 105 CFU of bacilli in lungs and BTLNs. Approximately 104 CFU of bacilli were observed in the liver and spleen. This level remained relatively constant by weeks 10-14. Then, the number of bacilli in the lungs increased to 106 CFU by week 22. Interestingly, the extent of bacterial colonization of BTLN and spleen decreased at around 14 weeks after infection. However, the bacilli burden of the spleen was elevated again at 22 weeks post-infection, while those of BTLN declined to 102 CFU by week 22. Therefore, the number of bacilli in BTLN was much less than that isolated from other organs. Overall, the pattern of bacterial growth in each organ of guinea pigs varied, similar to the pattern of cytokine gene expression.
Histopathological changes in response to M. tb infection were examined over the study period of 22 weeks after aerosol infection. At one week post-infection, which is the time of rapid multiplication of bacilli in the lungs, mild interstitial thickening due to the accumulation of inflammatory cells in the lungs were observed (Fig. 4). At 3 weeks post-infection, when the replication of bacilli reached peak levels, multifocal granuloma consisted of large numbers of alveolar macrophages surrounded by a mantle of lymphocytes, monocytes and occasionally epithelioid giant cells were observed and appeared around vessels. Caseous necrosis in the center of the lesions developed at 14 weeks post-infection, and the necrotic granuloma was confined by surrounding fibrous tissues (Fig. 4). At 22 weeks post-infection, there appeared diffuse granumatous bronchointerstitial pneumonia with mucopurulent bronchitis and granuloma inflammation that was composed of multinucleated giant cells, epithelioid cells and lymphocytes. The bacilli by acid-fast staining were detected in the granuloma of the lung at this time point.
Other organs such as BTLNs, spleens and livers were also examined. In BTLNs, like in the lungs, necrotic granuloma containing lymphocytes admixed with fibrous connective tissue developed at 14 weeks post-infection. At 6 weeks post-infection, spleen or liver showed severe and diffuse granuloma occupying the parenchyma of the tissues around the central arteries or in the portal area, respectively (Fig. 4).
Since immune responses to M. tb infection in human beings are highly diverse, the relationship between the pathogenesis and protective immune responses to TB cannot easily be examined in the human system. Guinea pig has been used as a gold standard for TB model because of its high susceptibility to M. tb infection and a number of differences on immune responses to mycobacterium have been identified compared to other animal models.14,20,22,35
When guinea pigs are infected with high dose of M. tb, they succumb shortly after the infection. Therefore, we applied low-dose aerosol M. tb infection to observe stepwise progress of disease development in this study. As illustrated in our results, M. tb bacilli were spread into each organ of the guinea pig by three weeks after infection. Gene expression profiles of both IFN-γ and TNF-α were induced in all four organs immediately following the increase of the M. tb load. Subsequently, the growth of M. tb in all four organs was contained and reached a stationary phase at around 14 weeks post-infection. The detection of IFN-γ and TNF-α gene expression suggests that their immune responses are efficiently induced at lesions formed by M. tb infection in guinea pigs. The maximal bacterial growth was about 1 log lower in liver than other organs and this lower number of bacilli growth seemed to induce lower levels of IFN-γ and TNF-α gene expression in liver compared to other organs.
We observed that the kinetic profiles of IFN-γ and TNF-α gene expression were different in each infected organ. Higher levels of IFN-γ gene expression was maintained in the lymph nodes until 22 weeks post-infection, while the number of bacilli in lymph nodes decreased during this period. This observation also indicates that the induction of IFN-γ expression is important in protecting guinea pigs against M. tb infection as the same phenomenon has been demonstrated in other studies.36,37 Meanwhile, the number of bacilli was increasing in other organs. In the lungs, the number of bacilli reached the stationary stage from 3 weeks and started to increase again in 22 weeks post-infection. During this period, we observed that the IFN-γ level was not induced steadily. Instead, expression of IFN-γ was decreased at 6 and 14 weeks post-infection and increased at 10 and 22 weeks post-infection in the lung, although bacterial load was persistently maintained. When the inhaled bacilli were replicating in the lung, the IFN-γ gene expression was effectively induced to control the bacilli replication up to 3 weeks post-infection. At around 6 weeks post-infection, the IFN-γ gene expression was decreased, probably due to the production of anti-inflammatory cytokines such as TGF-β which was triggered to counteract injurious inflammatory responses. Subsequently, the IFN-γ response was increased again at 10 weeks post-infection to down-regulate anti-inflammatory cytokines. At 14 and 22 weeks post-infection, this feedback mechanism of pro-inflammatory and anti-inflammatory responses seems to repeat again.38,39 In spleens, the number of bacilli increased again when the IFN-γ and TNF-α level decreased at around 14 weeks post-infection. As suggested, this result indicates that IFN-γ and TNF-α may act as one of the key factors to control TB infection in guinea pigs. Our observation also suggests the timely intervention of CMI to be an another important prospect for controlling the incurrence of active TB and dissemination.40
Consistent with the induction of IFN-γ and TNF-α, in our result, the granuloma formation was clearly observed in every organ regardless of whether the number of bacilli increased or decreased. However, this protective immunity was accompanied with progressive severe tissue damage. Particularly, the progression into necrotic granuloma was observed in the lungs at around week 14. Necrotic or diffuse granuloma also was observed in BTLNs, spleens and livers between 6 and 14 weeks post-infection. Therefore, this observation indicates that the expression of these type I cytokines did not efficiently inhibit the growth of M. tb or clearance of bacilli, resulting in persistent spread of the infection into the whole body, although the induction of IFN-γ and TNF-α may be able to prevent the continual multiplication of M. tb in each organ.
It has been known that the cytokine responses are altered during therapeutic treatment of TB patients. For example, the TNF-α, IFN-γ and IL-4 expression was observed in the lung biopsy samples of TB patients or in pulmonary granulomas of macaques infected with M. tb.41,42 On the contrary, the IFN-γ and TNF-α were not induced in lung lesions from pulmonary TB patients in other studies.32,43 These conflicting observations may indicate that the cytokine expression at the site of disease is stage-specific depending on the progress of disease or degree of pathogenesis.30 In addition, the expression of other cytokines such as TGF-β, IL-10, IL-1, IL-4 and IL-12 may be required to optimize the expression or activity of type I cytokines for favorable immune responses against TB.44-49
It has been suggested that T cell subsets inducing protective cell-mediated immune responses are different from those inducing DTH responses.31,50 Further studies on cellular composition and cytokine production together with histological analysis, in guinea pig model chronically infected with low dose infection, may help understand the mechanisms of pathogenesis, immune evasion and protection against TB. The availability of a suitable animal model, whose detailed mechanism in the development of TB is clearly comprehended, will eventually facilitate the development of vaccines and therapeutic drugs for TB.
Figures and Tables
Fig. 1
Total body weight of guinea pigs measured after an aerosol infection with Mycobacterium tuberculosis(M. tb). After infection with M. tb, guinea pigs gained less weight compared to the uninfected control. Body weight was calculated as the mean of weights of all infected guinea pigs per time (0 and 1 week: 9, 3 wk: 7, 6 wk: 5, 10 wk: 3, 14 wk: 2 and 22 wk: 1 animal) and only one animal chosen as an uninfected control was used in this graph. The statistical analysis was not performed since the size of experimental group varied at each time point.
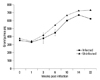
Fig. 2
TNF-α and IFN-γ synthesis in each organ of guinea pigs in response to M. tb infection. Changes in mRNA expression profiles of IFN-γ and TNF-α versus time course of infection with M. tb in each organ (lung, BTLN, spleen and liver) of guinea pigs were determined by RT-PCR analysis at each data point. (A) RT-PCR products were resolved on an agarose gel. (B) The gene expression of IFN-γ (white bar) or TNF-α (grey bar) was normalized with β-actin based on densitometry analysis. Each histogram represents mean±SD IFN-γ or TNF-α gene expression ratio to β-actin from two RT-PCR experiments. BTLN, bronchotracheal lymph node; M. tb, Mycobacterium tuberculosis.
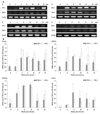
Fig. 3
Growth curves of M. tb in each organ. The M. tb growth in lungs, BTLNs, spleens and livers of guinea pigs were measured after an aerosol infection with about 106 colony forming units (CFUs) per exposure. The number of mean viable CFUs in each organ at different time intervals following the infection was calculated by plating the serial dilutions of homogenates of each organ on Middlebrook 7H11 agar and counting CFUs after 14-21 days. BTLN, bronchotracheal lymph node; M. tb, Mycobacterium tuberculosis.
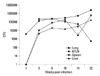
Fig. 4
Histopathological findings of uninfected or M. tb infected guinea pigs. (A-F) Lung (A) normal (40×), (B) at 1 week post-infection; mild interstitial thickening by the accumulation of inflammatory cells (40×), (C) at 3 weeks post-infection; multifocal granulomas composed of macrophages, epithelioid cells and lymphocytes develop (arrows) in the angiocentric area (40×). (D) At 14 weeks post-infection; necrotizing fibrous granulomas with caseous necrosis center of the lesion develops (arrows) (40×), (Ea and Eb) at 22 weeks post-infection (100×); bronchial tuberculosis and diffuse granulomatous inflammation are seen. (F) Acid-fast stain after 22 weeks, multiple red, rod-shaped bacilli are shown (arrows) (1000×). (G and H) BTLN. (G) Normal (40×), (H) severe necrotizing fibrous granuloma after 14 weeks (arrows) (100×). (I and J) Spleen. (I) Normal (40×), (J) the necrotic granuloma around the central arteries after 6 weeks (40×). (K and L) Liver. (K) Normal (40×), (L) the scattered granuloma in the portal area after 6 weeks (arrows) (40×). BTLN, bronchotracheal lymph node; M. tb, Mycobacterium tuberculosis.
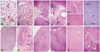
ACKNOWLEDGEMENTS
This work was supported partly by the National Research laboratory Program of the Ministry of Science and Technology (M1-0204-00-0146), the Brain Korea 21 Project for Medical Sciences in Yonsei University, and Priority Research Centers Program through the National Research Foundation of Korea (NRF) funded by the Ministry of Education, Science and Technology (2009-0093822).
References
1. Tuberculosis control and research strategies for the 1990s: memo-randum from a WHO meeting. Bull World Health Organ. 1992. 70:17–21.
2. Corbett EL, Watt CJ, Walker N, Maher D, Williams BG, Raviglione MC, et al. The growing burden of tuberculosis: global trends and interactions with the HIV epidemic. Arch Intern Med. 2003. 163:1009–1021.
3. WHO. Global tuberculosis report 2011. Available from: http://www.who.int/tb/publications/global_report/2011/en/index.html.
4. Roche PW, Triccas JA, Winter N. BCG vaccination against tuberculosis: past disappointments and future hopes. Trends Microbiol. 1995. 3:397–401.


5. Karonga Prevention Trial Group. Randomised controlled trial of single BCG, repeated BCG, or combined BCG and killed Mycobacterium leprae vaccine for prevention of leprosy and tuberculosis in Malawi. Lancet. 1996. 348:17–24.
6. McShane H. Tuberculosis vaccines: beyond bacille Calmette-Guerin. Philos Trans R Soc Lond B Biol Sci. 2011. 366:2782–2789.
7. Rowland R, McShane H. Tuberculosis vaccines in clinical trials. Expert Rev Vaccines. 2011. 10:645–658.


8. Shah NS, Wright A, Bai GH, Barrera L, Boulahbal F, Martín-Casabona N, et al. Worldwide emergence of extensively drug-resistant tuberculosis. Emerg Infect Dis. 2007. 13:380–387.


9. Chan ED, Iseman MD. Multidrug-resistant and extensively drug-resistant tuberculosis: a review. Curr Opin Infect Dis. 2008. 21:587–595.


10. Barry CE 3rd, Blanchard JS. The chemical biology of new drugs in the development for tuberculosis. Curr Opin Chem Biol. 2010. 14:456–466.


11. Shin SS, Keshavjee S, Gelmanova IY, Atwood S, Franke MF, Mishustin SP, et al. Development of extensively drug-resistant tuberculosis during multidrug-resistant tuberculosis treatment. Am J Respir Crit Care Med. 2010. 182:426–432.


12. Gandhi NR, Moll A, Sturm AW, Pawinski R, Govender T, Lalloo U, et al. Extensively drug-resistant tuberculosis as a cause of death in patients co-infected with tuberculosis and HIV in a rural area of South Africa. Lancet. 2006. 368:1575–1580.


13. Ma Z, Lienhardt C, McIlleron H, Nunn AJ, Wang X. Global tuberculosis drug development pipeline: the need and the reality. Lancet. 2010. 375:2100–2109.


14. Dannenberg AM Jr. Perspectives on clinical and preclinical testing of new tuberculosis vaccines. Clin Microbiol Rev. 2010. 23:781–794.


15. Cole ST, Riccardi G. New tuberculosis drugs on the horizon. Curr Opin Microbiol. 2011. 14:570–576.


16. Okada M, Kita Y. Tuberculosis vaccine development: the development of novel (preclinical) DNA vaccine. Hum Vaccin. 2010. 6:297–308.


17. Checkley AM, McShane H. Tuberculosis vaccines: progress and challenges. Trends Pharmacol Sci. 2011. 32:601–606.


18. Smith DW, Balasubramanian V, Wiegeshaus E. A guinea pig model of experimental airborne tuberculosis for evaluation of the response to chemotherapy: the effect on bacilli in the initial phase of treatment. Tubercle. 1991. 72:223–231.


19. Dannenberg AM Jr, Collins FM. Progressive pulmonary tuberculosis is not due to increasing numbers of viable bacilli in rabbits, mice and guinea pigs, but is due to a continuous host response to mycobacterial products. Tuberculosis (Edinb). 2001. 81:229–242.


20. McMurray DN, Allen SS, Jeevan A, Lasco T, Cho H, Skwor T, et al. Vaccine-induced cytokine responses in a guinea pig model of pulmonary tuberculosis. Tuberculosis (Edinb). 2005. 85:295–301.


21. Sugawara I, Udagawa T, Aoki T, Mizuno S. Establishment of a guinea pig model of latent tuberculosis with GFP-introduced Mycobacterium tuberculosis. Tohoku J Exp Med. 2009. 219:257–262.


23. Ahmad Z, Fraig MM, Pinn ML, Tyagi S, Nuermberger EL, Grosset JH, et al. Effectiveness of tuberculosis chemotherapy correlates with resistance to Mycobacterium tuberculosis infection in animal models. J Antimicrob Chemother. 2011. 66:1560–1566.


24. Grover A, Troudt J, Arnett K, Izzo L, Lucas M, Strain K, et al. Assessment of vaccine testing at three laboratories using the guinea pig model of tuberculosis. Tuberculosis (Edinb). 2012. 92:105–111.


25. Salgame P. Host innate and Th1 responses and the bacterial factors that control Mycobacterium tuberculosis infection. Curr Opin Immunol. 2005. 17:374–380.


27. Jeon BY, Eoh H, Ha SJ, Bang H, Kim SC, Sung YC, et al. Co-immunization of plasmid DNA encoding IL-12 and IL-18 with Bacillus Calmette-Guérin vaccine against progressive tuberculosis. Yonsei Med J. 2011. 52:1008–1015.


28. North RJ, Jung YJ. Immunity to tuberculosis. Annu Rev Immunol. 2004. 22:599–623.
29. Gupta A, Kaul A, Tsolaki AG, Kishore U, Bhakta S. Mycobacterium tuberculosis: immune evasion, latency and reactivation. Immunobiology. 2012. 217:363–374.


30. Brighenti S, Andersson J. Local immune responses in human tuberculosis: learning from the site of infection. J Infect Dis. 2012. 205:Suppl 2. S316–S324.


31. Orme IM, Cooper AM. Cytokine/chemokine cascades in immunity to tuberculosis. Immunol Today. 1999. 20:307–312.


32. Rahman S, Gudetta B, Fink J, Granath A, Ashenafi S, Aseffa A, et al. Compartmentalization of immune responses in human tuberculosis: few CD8+ effector T cells but elevated levels of FoxP3+ regulatory t cells in the granulomatous lesions. Am J Pathol. 2009. 174:2211–2224.
33. Kawahara M, Nakasone T, Honda M. Dynamics of gamma interferon, interleukin-12 (IL-12), IL-10, and transforming growth factor beta mRNA expression in primary Mycobacterium bovis BCG infection in guinea pigs measured by a real-time fluorogenic reverse transcription-PCR assay. Infect Immun. 2002. 70:6614–6620.


34. Cho H, Lasco TM, Allen SS, Yoshimura T, McMurray DN. Recombinant guinea pig tumor necrosis factor alpha stimulates the expression of interleukin-12 and the inhibition of Mycobacterium tuberculosis growth in macrophages. Infect Immun. 2005. 73:1367–1376.


35. McMurray DN. Hematogenous reseeding of the lung in low-dose, aerosol-infected guinea pigs: unique features of the host-pathogen interface in secondary tubercles. Tuberculosis (Edinb). 2003. 83:131–134.


36. Cooper AM, Dalton DK, Stewart TA, Griffin JP, Russell DG, Orme IM. Disseminated tuberculosis in interferon gamma gene-disrupted mice. J Exp Med. 1993. 178:2243–2247.


37. Flynn JL, Chan J, Triebold KJ, Dalton DK, Stewart TA, Bloom BR. An essential role for interferon gamma in resistance to Mycobacterium tuberculosis infection. J Exp Med. 1993. 178:2249–2254.


38. Ly LH, Russell MI, McMurray DN. Cytokine profiles in primary and secondary pulmonary granulomas of Guinea pigs with tuberculosis. Am J Respir Cell Mol Biol. 2008. 38:455–462.


39. Ly LH, Russell MI, McMurray DN. Microdissection of the cytokine milieu of pulmonary granulomas from tuberculous guinea pigs. Cell Microbiol. 2007. 9:1127–1136.


40. Chackerian AA, Alt JM, Perera TV, Dascher CC, Behar SM. Dissemination of Mycobacterium tuberculosis is influenced by host factors and precedes the initiation of T-cell immunity. Infect Immun. 2002. 70:4501–4509.


41. Fenhalls G, Stevens L, Bezuidenhout J, Amphlett GE, Duncan K, Bardin P, et al. Distribution of IFN-gamma, IL-4 and TNF-alpha protein and CD8 T cells producing IL-12p40 mRNA in human lung tuberculous granulomas. Immunology. 2002. 105:325–335.


42. Fuller CL, Flynn JL, Reinhart TA. In situ study of abundant expression of proinflammatory chemokines and cytokines in pulmonary granulomas that develop in cynomolgus macaques experimentally infected with Mycobacterium tuberculosis. Infect Immun. 2003. 71:7023–7034.


43. Aung H, Toossi Z, McKenna SM, Gogate P, Sierra J, Sada E, et al. Expression of transforming growth factor-beta but not tumor necrosis factor-alpha, interferon-gamma, and interleukin-4 in granulomatous lung lesions in tuberculosis. Tuber Lung Dis. 2000. 80:61–67.


44. Dai G, McMurray DN. Effects of modulating TGF-beta 1 on immune responses to mycobacterial infection in guinea pigs. Tuber Lung Dis. 1999. 79:207–214.


45. Fenhalls G, Wong A, Bezuidenhout J, van Helden P, Bardin P, Lukey PT. In situ production of gamma interferon, interleukin-4, and tumor necrosis factor alpha mRNA in human lung tuberculous granulomas. Infect Immun. 2000. 68:2827–2836.


46. Allen SS, Cassone L, Lasco TM, McMurray DN. Effect of neutralizing transforming growth factor beta1 on the immune response against Mycobacterium tuberculosis in guinea pigs. Infect Immun. 2004. 72:1358–1363.


47. Grover A, Taylor J, Troudt J, Keyser A, Arnett K, Izzo L, et al. Kinetics of the immune response profile in guinea pigs after vaccination with Mycobacterium bovis BCG and infection with Mycobacterium tuberculosis. Infect Immun. 2009. 77:4837–4846.


48. Jeevan A, Yoshimura T, Ly LH, Dirisala VR, McMurray DN. Cloning of guinea pig IL-4: reduced IL-4 mRNA after vaccination or Mycobacterium tuberculosis infection. Tuberculosis (Edinb). 2011. 91:47–56.

