Abstract
Purpose
Endothelial cells maintain the homeostasis of blood, which consists of plasma and cellular components, and regulate the interaction between blood and the surrounding tissues. They also have essential roles in vascular permeability, the circulation, coagulation, inflammation, wound healing, and tissue growth. The senescence of endothelial cells is closely related to the aging of the adjacent tissues and to age-related vascular disease. Recently, the expression of moesin was found to be decreased in elderly human dermal microvascular endothelial cells (HDMECs), and an association between moesin and senescence has been suggested. This study examined the functional role of moesin in cellular senescence.
Materials and Methods
To study the effects of decreased moesin expression on cellular senescence and metabolism, HDMECs were transfected with short hairpin-RNA (shRNA) lentivirus to silence moesin gene expression. In addition, specimens from young and old human skin were stained with anti-moesin and anti-p16 antibodies as an in vivo study.
Results
Using shRNAl-entivirus, moesin knock-down HDMECs developed characteristics associated with aging and expressed senescence associated-beta-galactosidase during early passages. They also showed increased p16 expression, decreased metabolic activity, and cell growth retardation. Human skin tissue from elderly persons showed decreased moesin expression and increased p16 expression.
Conclusion
These findings suggest that there is a functional association between moesin expression and cellular senescence. Further study of the functional mechanism of moesin in the cytoskeleton and cellular senescence is needed. In addition, this study provides a useful model for developing anti-aging treatments.
To understand skin aging, studies need to examine the major cutaneous components, including keratinocytes, fibroblasts, and vascular endothelial cells. Cellular senescence is accelerated by diverse stresses, and various factors involved in cell senescence have been reported. For example, telomere uncapping, DNA damage, oxidative stress, oncogene activation, nutritional deficiency, growth factor deficiency, and mitochondrial damage all play roles.1,2 Protein expression is altered in aging cells and has an important role in the pathogenesis of aging. The expression of insulin-like growth factor binding protein-5, neurofilament subunit L, adenosine A2A receptor, plasminogen activator inhibitor-1, intercellular adhesion molecule-1, p16, and p27 is altered in senescent endothelial cells.3-6
The vascular endothelial cells between blood and tissues maintain homeostasis through their interaction with plasma. They have the capacity to generate new blood vessels and to maintain their integrity, and their normal function is important for inflammatory reactions, coagulation, wound healing, and tissue growth.7,8 The senescence of vascular endothelial cells is associated with atherosclerosis, hypertension, congestive heart failure, sepsis, diabetes, thrombotic thrombocytopenic purpura, systemic sclerosis, systemic lupus erythematosus, and the metastasis of cancer, as well as the intrinsic aging of the skin.9-18 However, the precise mechanisms of senescence have not been elucidated. The growth, aging, and apoptosis of vascular endothelial cells can be induced or suppressed in response to stimulation by serum factors or adjacent cells. Since most pathophysiological conditions occur at the level of the microvasculature, endothelial cells are very suitable as a research model for the aging of skin, prompting us to use these cells. Previously, our studies have shown that kinetin, epigallocatechin-3-gallate, all-trans retinoic acid, and selenium have anti-aging effects on cultured human dermal microvascular endothelial cells (HDMECs).19,20 Using these materials, several proteins suspected of being aging-related proteins were detected in proteomic maps of HDMECs obtained before and after exposure to anti-oxidants.20 Moesin, Rho guanosine-5'-diphosphate-dissociation inhibitor, and actin played significant roles, especially moesin.
Moesin is an ezrin/radixin/moesin (ERM) protein and belongs to the band 4.1 superfamily. Members of the band 4.1 superfamily share a 300-amino-acid domain called the 4.1 ezrin, radixin, and moesin (FERM) domain.21-23 Moesin acts as a cytoskeleton protein connecting cell membrane proteins and actins located underneath the cell membrane. It maintains cell polarity or integrity and regulates the functions of cell membrane proteins. It also has a signal transduction function in addition to its role in remodeling the cytoskeleton.24-26 Recently, it was reported to be associated with conjugation between T cells and antigen-presenting cells, leukocyte diapedesis, and endothelial cell migration and permeability.27-29 It has been suggested as a potential prognostic marker of thyroid cancer, renal cell carcinoma, and pancreatic adenocarcinoma.30-32 Ezrin induces apoptosis via Fas,33 and Gα13 activation rescues moesin-depletion-induced apoptosis in F9 teratocarcinoma cells.34 These findings suggest that ERM proteins are closely related to apoptosis and aging.
To examine the functional role of moesin in skin aging, we examined the effect of moesin on aging and metabolic function using a short hairpin-RNA (shRNA) lentivirus to suppress moesin expression in subcultured HDMECs. In addition, the difference in moesin and p16 expression in young and old skin tissues was examined.
Human dermal microvascular endothelial cells were purchased from Cambrex (Walkersville, MA, USA). HDMECs were prepared and treated in a tissue incubator with 0.1% gelatin, using microvascular endothelial cell medium-2 (Cambrex) containing human epidermal growth factor, hydrocortisone acetate, vascular endothelial growth factor, human fibroblast growth factor-B, gentamicin, amphotericin B, 5% fetal bovine serum, R3-insulin growth factor-1, and ascorbic acid. They were subcultured serially from passage 5 to 25 at 37℃ in a CO2 incubator.
A shRNA-lentiviral vector targeting moesin was prepared by inserting a synthetic double-stranded oligonucleotide (5'-GCAGCGCATTGACGAATTTGA-3') in the EcoRI-XbaI restriction enzyme site of shLenti2.4R lentiviral vector (VectorCore A, Daejeon, Korea). The shLenti2.4R lentiviral vector was designed to express shRNA at the human U6 promoter and to express red fluorescent protein (RFP) from the hCMV promoter. A shLenti2.4R lentiviral vector with a scrambled shRNA (5'-AATCGCATTAGCGTATGCCGTT-3') insert was used as the control vector. The scrambled shRNA lacks homology with any mammalian mRNA sequence.
The recombinant lentiviral vector was manufactured by VectorCore A. 293T cells were transfected with transfer vector, VSV-G expression vector, and gag-pol expression vector in a 1 : 1 : 1 ratio using LipofectAMINE PLUS (Invitrogen, Carlsbad, CA, USA). The supernatant of the culture medium containing the lentiviral vector was collected 48 h after transfection and was refined using 0.45-µm membrane filters (Nalgene, Rochester, NY, USA).
Phase-contrast microscopy (Olympus, Tokyo, Japan) was used to confirm the senescence of HDMECs and to examine the morphologic changes of transfected HDMECs according to the number of serial passages.
The modified senescence-associated-β-galactosidase (SA-β-gal) assay was used to compare senescence according to the number of serial passages of untreated HDMECs, control vector-treated HDMECs, and moesin-knock-down HDMECs. The cells were washed twice with phosphate-buffered saline (PBS), fixed in 3% (v/v) formaldehyde for 3 min at room temperature, washed again in PBS, and incubated for 12 h with 500 µL of β-GAL solution containing 5-bromo-4-chloro-3-indolyl-β-D-galactoside (X-gal), 5 mM potassium ferrocyanide, 150 nM NaCl, and 2 mM MgCl2. The cells were viewed and photographed with a light microscope fitted with a digital camera.
To obtain lifespan curves, cell cultures were serially passaged until the end of their proliferative capacity in vitro and the population-doubling levels were determined. The time required for 1×105 HDMECs to double was regarded as the doubling time.
Virus-untreated HDMECs, HDMECs over passage 25, control vector-treated HDMECs, and moesin-knock-down HDMECs were serially cultured. Total RNA was isolated using an RNeasy Mini Kit (QIAGEN, Valencia, CA, USA) according to the manufacturer's instructions. The RNA concentration was measured at 260 nm using an UV-1601 PC spectrophotometer (Shimadzu, Kyoto, Japan).
cDNA was synthesized from 1 µg of extracted total RNA using AccuPower RT PreMix (Bioneer, Seoul, Korea) and a PCR system 2700 (Applied Biosystems, Singapore, Singapore).
Polymer chain reaction amplification was carried out using 1 µg of cDNA as the template in 20 µL of PCR mixture containing 1 µL of 20 pmole primer with AccuPower RT PreMix (Bioneer). The PCR fragments were visualized on 2% agarose gels containing 5 µg/mL ethidium bromide. The same amount of actin was used as a standard for quantification. The moesin primers were 5'-CCACCATGCCCAAAACGATC-3' (forward) and 5'-GGTGCCCATTACATAGACTC-3' (reverse). The p16 primers were 5'-AGCATGGAGCCTTCGGCTGAC-3' (forward) and 5'-CTGTAGGACCTTCGGTGACTGAT-3' (reverse).
Immunoblotting was used to examine the moesin and p16 expression. Cells were transferred to a microcentrifuge tube and centrifuged for 10 min at 10,000 × g and 4℃. The supernatant was collected, and all of the supernatant was used as the cell lysate. After sodium dodecyl sulfate-polyacrylamide gel electrophoresis (SDS-PAGE), the proteins were transferred to an nitrocellulose (NC) membrane in a container filled with transfer buffer solution for 2 h at 400 mA and 10-15 V. The membrane was blocked with 5% blotting grade non-fat dry milk (Bio-Rad Laboratories, Hercules, CA, USA) for 60 min. Primary antibodies to moesin, p16, and actin (Santa Cruz Biotechnology, Santa Cruz, CA, USA) were diluted to 200 µg/mL in blocking solution and added to the membranes, and the mixture was incubated for 60 min. Antibody to horseradish peroxidase (HRP; Santa Cruz Biotechnology) diluted to 1 : 2,000 in blocking buffer solution was added as the secondary antibody, and reacted with the primary antibody for 60 min. The membrane was washed three times with TBST buffer solution for 5 min, and stained with 3,3,5,5-tetramethylbenzidine.
The tetrazolium salt MTT was used to compare the cellular metabolic capacities. Each well of a 96-well plate was seeded with 1×105 HDMECs and preincubated at 37℃ in a CO2 incubator. The culture medium was removed and the cells were incubated for 3 h with 200 µL of 0.5 mg/mL MTT (Boehringer, Mannheim, Germany). Metabolically active cells reduce the dye to purple formazan. The formazan crystals dissolved with 200 µL of dimethyl sulfoxide (DMSO; Sigma), and the absorbance was measured on an ELISA reader (Bio-Rad Laboratories), using a reference wavelength of 630 nm and a test wavelength of 570 nm.
To examine the expression of moesin and p16 in skin tissues, biopsies of the prepuce of neonates and the normal abdominal skin of a 10-year-old child and an 86-year-old adult were obtained. The neonatal prepuce was obtained from circumcision, and the abdominal skin was obtained with a 3-mm punch. The specimens were stored in Tissue-Tek (Sakura Finetechnical, Tokyo, Japan) at -70℃.
The stored tissues were cryosectioned at 6 µm and attached to silane-coated slides (Muto Pure Chemicals, Tokyo, Japan). They were fixed in acetone for 15 min and washed with PBS. Immunohistochemical staining was performed using the Histostatin-DS kit (Zymed Laboratories, San Francisco, CA, USA). To suppress nonspecific binding, the samples were placed in a blocking solution for 10 min. Mouse anti-human moesin monoclonal antibodies (GeneTex, San Antonio, TX, USA) diluted 1 : 25 with PBS containing 5% bovine serum and anti-human CD31 monoclonal antibodies (BD Biosciences, San Jose, CA, USA) were used and incubated at 4℃. The biotinylated secondary antibody was incubated for 10 min at room temperature. Streptavidin alkaline phosphatase (AP) conjugate was incubated for 10 min and nitroblue tetrazolium solution (NBT) was used as a substrate for the chromogen. For double immunohistochemical stains, double staining enhancer solution was added and treated for 30 min at room temperature. This procedure was then repeated with streptavidin horseradish peroxidase (HRP) instead of AP conjugate and 3-amino-9-ethylcarbazole (AEC) solution was used as the substrate for the chromogen.
At passage 6, HDMECs were transfected with shRNA-lentivirus, which knocked-down moesin expression, and RFP expression was examined to confirm whether the HDMECs had been transfected by the viral vector. Control vector-treated HDMECs and shRNA-lentivirus-treated HDMECs continuously showed RFP expression through passages 10 and 20 (Fig. 1).
Using contrast microscopy, moesin knock-down HDMECs were compared with virus-untreated HDMECs, HDMECs at passages higher than passage 25, and control vector treated HDMECs. A smaller degree of change in cell size and morphology was detected between passages 6 and 20 in virus-untreated HDMECs and control vector-treated HDMECs compared to moesin knock-down HDMECs at early and late passages. By contrast, moesin knock-down HDMECs showed the characteristics of aging, i.e., they were large, flat, and stellate, beginning at the early stage of passage 6.
Control vector-treated HDMECs stained with SA-β-gal were detected at passage 12. Moesin knock-down HDMECs from the early stage of passage 6 were stained blue with SA-β-gal. HDMECs at late passages higher than passage 25 stained blue in all serial subcultures (Fig. 2).
The growth of HDMECs was observed through lifespan curves over time in the cultures. Within the culture period, all HDMEC cultures showed an initial proliferation phase and late growth retardation phase. In comparison with control vector-treated HDMECs, the doubling time of moesin knock-down HDMECs was delayed by 3 h at passage 6 and 10 h at passage 22 (Fig. 3A). The doubling level of the cumulative cell population in moesin-knock-down HDMECs was much less than that in virus-untreated HDMECs (Fig. 3B).
The expression of moesin and p16 in cells was observed using RT-PCR and Western blotting. Moesin knock-down HDMECs expressed less moesin RNA than control vector-treated HDMECs (Fig. 4A). In addition, the RNA expression of p16 in HDMECs at late passages and moesin knock-down HDMECs was higher than that in control vector-treated HDMECs (Fig. 4B).
In applying Western blotting, reduced expression of moesin and higher expression of p16 were observed in moesin knock-down HDMECs, compared to control vector-treated HDMECs (Fig. 4C).
The MTT assay was performed in HDMECs at passage 20 to compare cell metabolic activity. Compared with control vector-treated HDMECs, cellular metabolic activity decreased in HDMECs at passage 39 and moesin-knock-down HDMECs (p = 0.012 and 0.016, respectively) (Fig. 5).
The neonatal prepuce and the skin of the 10-year-old child stained well with moesin, whereas the skin of the 86-year-old adult did not stain. Conversely, cells stained with p16 were not detected in the neonatal prepuce or the skin of the 10-year-old child, while the skin of the 86-year-old contained many cells stained with p16 (Fig. 6A). To exclude the influence of the distribution of blood vessels between the abdominal skin of the child and elderly adult on staining with moesin and p16, double staining with moesin/CD31 and with p16/CD31 was performed. The abdominal skin of the 10-year-old child and 86-year-old adult showed comparable CD31-positive cells and tissues (Fig. 6B).
With aging, the function of organs decreases gradually with cellular senescence. The replicative senescence of cells resulting in the loss of replication capacity and a change in the function and morphology of cells may be a key to research on the pathophysiology of aging.35
Ezrin, radixin, and moesin constitute the ERM protein family. ERM proteins are highly conserved throughout evolution. More than 75% identity is observed in the FERM domain and F-actin binding site of vertebrates (ezrin, radixin, and moesin), Drosophila (Dmoesin), and C. elegans (ERM-1).21,22 ERM proteins are essential for the morphogenesis of the apical domain of different epithelial cell types and regulate the functions of membrane proteins.23,24,32 Ezrin is part of a complex that regulates the Na+/H+ exchanger NHE3 in intestinal and renal brush border microvilli36 and influences transport through cellular membrane proteins.37 Dmoesin in Drosophila has a role in the physiological functions of photoreceptors by interacting with transient receptor potential (TRP) protein.38 Phagosome maturation in macrophages and dendritic cells depends on the activation of Rho via Rho kinase on ERM proteins,39 and ERM proteins are important downstream and upstream effectors of Rho GTPase, which is involved in phagosome maturation and phagocyte movement toward a dying cell.27,40 Few studies have examined the role of moesin in cellular senescence, although the functional role of moesin in cellular aging has been suggested from a number of reports, which have found that ERM protein induces Fasmediated apoptosis,33 and that the apoptosis of cancer cells was induced by the decrease in moesin expression.34 Therefore, we characterized the function of moesin by knocking out the moesin gene using shRNA.
RNA interference (RNAi) has become a widely used technique for silencing gene expression. Successful application of RNAi in mammalian cells depends on knocking-down targeted transcripts and the effective intracellular delivery of either preformed short interfering RNAs (siRNAs)/shRNAs or vector expressing si/shRNAs. Chemically synthesized siRNAs are incorporated into the RNA-induced silencing complex and the delivery results in sequence-specific silencing of the expression of the corresponding gene.41 RNAi can also be induced by the endogenous expression of shRNAs, which are structurally related to a highly conserved class of small RNAs known as microRNAs (miRNAs) that mediate RNAi through a translational inhibition mechanism.42 We used shRNAs for RNAi because knock-down by siRNAs was not maintained during the next passage. In contrast, we confirmed that knock-down by shRNA-lentiviral vector was maintained until passage 22 using RFP expression.
Contrast microscopy and the SA-β-gal assay were used to assess the senescence of vascular endothelial cells. SA-β-gal staining is widely used as a marker of senescence and its usefulness has been reported in human vascular endothelial cells, keratinocytes, fibroblasts, and smooth muscle cells.43,44 Nonetheless, we did not perform the SA-β-gal assay in tissue specimens because it has been reported that SA-β-gal stains have low usefulness in vivo.45 The early appearance of senescent cells was observed in moesin knock-down HDMECs using contrast microscopy and SA-β-gal staining. These cells were clearly different from the virus-untreated HDMECs. The HDMECs treated with scrambled shRNA-lentivirus also showed some changes of senescence. These might have been induced by the virus itself or the reagents used during viral transfection.
The doubling time of moesin knock-down HDMECs was prolonged and growth retardation was detected in the early phase in the life span curve. In addition, cellular metabolic activity decreased in moesin knock-down HDMECs. These findings show that moesin knock-down makes HDMECs senescence not only morphologically but also functionally, and that moesin is a potential biomarker of cellular aging of the skin.
Until now, the suggested aging mechanisms of normal cells have included cellular proliferation, oxidative stress, DNA damage, shortening of the telomere related to tumor suppressor cascades, p16INK4A, and the ARF-p53 pathway.46,47 It has been reported that cellular senescence progresses via p16 activation in response to oxidative stress or telomere shortening rather than the ARF pathway.1,48 We showed that p16 expression increased with cell aging, but further studies need to examine whether telomere shortening is associated with p16 and whether moesin is associated with p19ARF and the p53 pathway via direct DNA. It has also been reported that oxidative stress activates p16 via the stress-activated protein kinase family, p38-MAPK;49 therefore, a study of p38-MAPK is needed to determine whether moesin is related to the oxidative stress associated pathway. The mitochondria play a major role in the production of reactive oxygen species, and the accumulation of mutated mitochondrial DNA has also been proposed as a cause of cellular aging.2,50 We also need to study the association between moesin and mitochondria in the cell cycle and senescence.
Cell senescence or apoptosis is closely related to the cell cycle: if cellular senescence occurs, G1 arrest occurs, and the G1-specific cyclin D1 or cyclin E1, pRB, p16, p21, and p27 undergo change.6 p16 maintains Rb in its hypophosphorylated form and hypophosphorylated Rb suppresses E2F activation and CDK4/6. Consequently, p16 induces cell cycle arrest.51,52 Based on the increased p16 expression in cell senescence, we postulate that the cellular aging caused by moesin knock-down is associated with p16, because p53 and p21 are involved in aging through mechanisms involving DNA damage. Since p16INK4A expression is directly correlated with the chronological aging of human skin in vivo, and is a biomarker for human aging in vivo,53 immunohistochemical staining of p16 could be used in studies of skin aging.
To determine the function of moesin, experimental methods could involve the suppression or overexpression of moesin. However, since the overexpression of moesin is anticipated to be associated with oncogenesis in addition to anti-aging, the effect of moesin suppression on the aging of cells was investigated first. We postulate that the selective suppression of moesin in cancer cells may have an anti-cancer effect; indeed, moesin expression is enhanced in cancer cells and it has been suggested as a potential prognostic and diagnostic marker of renal cell carcinoma.30,54,55 In addition, moesin-knock-down with shRNA has been reported to induce the apoptosis of teratocarcinoma cells.34 Therefore, the possibility of tumor development via moesin overexpression should be examined in future experiments.
Our study showed that the signs of cellular senescence appeared early with moesin knock-down, and that cell cycle arrest occurred on inducing the increased expression of p16. Moesin was postulated to influence cell senescence, and it should be a useful marker for cell senescence, as well as for evaluating the level of aging. If the acceleration of aging were proven in a moesin knock-down animal model, it could be a breakthrough model for aging experiments, and could be used to validate the efficacy of anti-aging treatments. Furthermore, it could be very useful to studies on the pathophysiological mechanism of various diseases of aging, as long as the factors and mechanisms influencing moesin expression can be determined.
Figures and Tables
Fig. 1
Confirming the infection of HDMEC by shRNA-lentivirus. Control vector-treated HDMECs and shRNA-lentivirus-treated HDMECs showed RFP expression at p6, p10, and p20. HDMEC, human dermal microvasular endothelial cell; shRNA, short hairpin-RNA; CTR, control; CTR-V, control-virus; RFP, red fluorescent protein.
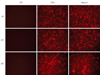
Fig. 2
Comparison of senescent level in HDMEC transfected with shRNA-lentivirus during subculture. Control vector-treated HDMECs without moesin knock-down (CTR-V) from passage 12, moesin knock-down HDMECs (Moesin-V) from passage 6, and HDMEC at over passage 25 (CTR-Aged) were stained as blue with SA-β-gal staining. HDMEC, human dermal microvasular endothelial cell; SA-β-gal, senescence-associated-β-galactosidase.
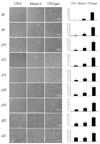
Fig. 3
Doubling time and doubling level of cumulative cell population. (A) The doubling time of Moesin-V group was longer than those of CTR-V group at passage 6 to 22. (B) Doubling level of Moesin-V group was much less than that of CTR or CTR-V group. Error bars indicates standard deviations. These experiments have been performed in a pair three times.
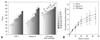
Fig. 4
Expression of moesin/p16 RNA and protein. (A) Moesin RNA expression in Moesin-V group and CTR-Aged group was lower than that in CTR-V group. (B) In comparison with CTR-V group, CTR-Aged and Moesin-V groups showed higher RNA expression of p16. (C) Applying Western blotting, reduced expression of moesin and higher expression of p16 were observed in Moesin-V group, compared to CTR-V group at passage 14. Left panels, autoradiographs of mRNA or protein levels; right panels, relative amounts of mRNA or protein as estimated by an imaging analyzer. The values obtained were normalized by β-actin mRNA or protein content. Error bars indicates standard deviations. These experiments have been performed in a pair three times.
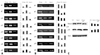
Fig. 5
Comparison of cellular metabolic activity. Cellular metabolic activity was measured by MTT assay (48 hours). Cellular metabolic activity significantly decreased in CTR-Aged group (passage 39) and Moesin V group compared to CTR-V group (*p = 0.016, **p = 0.012). Mann-Whitney U-test was performed for statistical analysis. Error bars indicates standard deviations. These experiments have been performed in a pair three times.
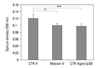
Fig. 6
Immunohistochemical staining of moesin and p16. (A) Immunohistochemical staining of moesin and p16 was performed on the neonatal prepuce (Age 1) and the skin of the 10-year-old child (Age 10) and the 86-year-old adult (Age 86). Moesin was stained well as pink or brown in Age 1 and Age 10, on the other hand, moesin was not detected well in Age 86. In contrast, p16 stained cells were not detected well in Age 1 and Age 10 and numerous p16 stained cells were observed in Age 86. (B) Comparable CD31 positive cells and tissues were observed at double staining of moesin/CD31 and p16/CD31 between Age 10 and Age 86. Double stained cells were observed as purple.
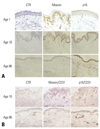
ACKNOWLEDGEMENTS
This work was supported by a grant of the Korea Health 21 R&D Project, Ministry of Health & Welfare, Republic of Korea (A030003).
References
1. Ben-Porath I, Weinberg RA. The signals and pathways activating cellular senescence. Int J Biochem Cell Biol. 2005. 37:961–976.


2. Kujoth GC, Hiona A, Pugh TD, Someya S, Panzer K, Wohlgemuth SE, et al. Mitochondrial DNA mutations, oxidative stress, and apoptosis in mammalian aging. Science. 2005. 309:481–484.


3. Shelton DN, Chang E, Whittier PS, Choi D, Funk WD. Microarray analysis of replicative senescence. Curr Biol. 1999. 9:939–945.


4. Comi P, Chiaramonte R, Maier JA. Senescence-dependent regulation of type 1 plasminogen activator inhibitor in human vascular endothelial cells. Exp Cell Res. 1995. 219:304–308.


5. Tang J, Gordon GM, Nickoloff BJ, Foreman KE. The helix-loophelix protein id-1 delays onset of replicative senescence in human endothelial cells. Lab Invest. 2002. 82:1073–1079.


6. Wagner M, Hampel B, Bernhard D, Hala M, Zwerschke W, Jansen-Dürr P. Replicative senescence of human endothelial cells in vitro involves G1 arrest, polyploidization and senescence-associated apoptosis. Exp Gerontol. 2001. 36:1327–1347.


8. Cines DB, Pollak ES, Buck CA, Loscalzo J, Zimmerman GA, McEver RP, et al. Endothelial cells in physiology and in the pathophysiology of vascular disorders. Blood. 1998. 91:3527–3561.
9. Hasdai D, Sangiorgi G, Spagnoli LG, Simari RD, Holmes DR Jr, Kwon HM, et al. Coronary artery apoptosis in experimental hypercholesterolemia. Atherosclerosis. 1999. 142:317–325.


10. Haunstetter A, Izumo S. Apoptosis: basic mechanisms and implications for cardiovascular disease. Circ Res. 1998. 82:1111–1129.
11. Singhal PC, Gibbons N, Franki N, Reddy K, Sharma P, Mattana J, et al. Simulated glomerular hypertension promotes mesangial cell apoptosis and expression of cathepsin-B and SGP-2. J Investig Med. 1998. 46:42–50.
12. Vescovo G, Zennaro R, Sandri M, Carraro U, Leprotti C, Ceconi C, et al. Apoptosis of skeletal muscle myofibers and interstitial cells in experimental heart failure. J Mol Cell Cardiol. 1998. 30:2449–2459.
13. Galley HF, Howdle PD, Walker BE, Webster NR. The effects of intravenous antioxidants in patients with septic shock. Free Radic Biol Med. 1997. 23:768–774.
14. Mizutani M, Kern TS, Lorenzi M. Accelerated death of retinal microvascular cells in human and experimental diabetic retinopathy. J Clin Invest. 1996. 97:2883–2890.


15. Sgonc R, Gruschwitz MS, Dietrich H, Recheis H, Gershwin ME, Wick G. Endothelial cell apoptosis is a primary pathogenetic event underlying skin lesions in avian and human scleroderma. J Clin Invest. 1996. 98:785–792.


16. Dang CT, Magid MS, Weksler B, Chadburn A, Laurence J. Enhanced endothelial cell apoptosis in splenic tissues of patients with thrombotic thrombocytopenic purpura. Blood. 1999. 93:1264–1270.


17. Lai KN, Leung JC, Lai KB, Lai CK. Effect of anti-DNA autoantibodies on the gene expression of interleukin 8, transforming growth factor-beta, and nitric oxide synthase in cultured endothelial cells. Scand J Rheumatol. 1997. 26:461–467.
18. Kebers F, Lewalle JM, Desreux J, Munaut C, Devy L, Foidart JM, et al. Induction of endothelial cell apoptosis by solid tumor cells. Exp Cell Res. 1998. 240:197–205.
19. Ha MK, Soo Cho J, Baik OR, Lee KH, Koo HS, Chung KY. Caenorhabditis elegans as a screening tool for the endothelial cell-derived putative aging-related proteins detected by proteomic analysis. Proteomics. 2006. 6:3339–3351.
20. Lee JH, Chung KY, Bang D, Lee KH. Searching for aging-related proteins in human dermal microvascular endothelial cells treated with anti-aging agents. Proteomics. 2006. 6:1351–1361.


21. Chishti AH, Kim AC, Marfatia SM, Lutchman M, Hanspal M, Jindal H, et al. The FERM domain: a unique module involved in the linkage of cytoplasmic proteins to the membrane. Trends Biochem Sci. 1998. 23:281–282.
22. Bretscher A, Edwards K, Fehon RG. ERM proteins and merlin: integrators at the cell cortex. Nat Rev Mol Cell Biol. 2002. 3:586–599.


23. Fiévet B, Louvard D, Arpin M. ERM proteins in epithelial cell organization and functions. Biochim Biophys Acta. 2007. 1773:653–660.
24. Tsukita S, Yonemura S. ERM (ezrin/radixin/moesin) family: from cytoskeleton to signal transduction. Curr Opin Cell Biol. 1997. 9:70–75.
26. Polesello C, Payre F. Small is beautiful: what flies tell us about ERM protein function in development. Trends Cell Biol. 2004. 14:294–302.


27. Charrin S, Alcover A. Role of ERM (ezrin-radixin-moesin) proteins in T lymphocyte polarization, immune synapse formation and in T cell receptor-mediated signaling. Front Biosci. 2006. 11:1987–1997.


28. Ivetic A, Ridley AJ. Ezrin/radixin/moesin proteins and Rho GTPase signalling in leucocytes. Immunology. 2004. 112:165–176.


29. Simoncini T, Scorticati C, Mannella P, Fadiel A, Giretti MS, Fu XD, et al. Estrogen receptor alpha interacts with Galpha13 to drive actin remodeling and endothelial cell migration via the RhoA/Rho kinase/moesin pathway. Mol Endocrinol. 2006. 20:1756–1771.
30. Brown LM, Helmke SM, Hunsucker SW, Netea-Maier RT, Chiang SA, Heinz DE, et al. Quantitative and qualitative differences in protein expression between papillary thyroid carcinoma and normal thyroid tissue. Mol Carcinog. 2006. 45:613–626.


31. Craven RA, Stanley AJ, Hanrahan S, Dods J, Unwin R, Totty N, et al. Proteomic analysis of primary cell lines identifies protein changes present in renal cell carcinoma. Proteomics. 2006. 6:2853–2864.
32. Torer N, Kayaselcuk F, Nursal TZ, Yildirim S, Tarim A, Nòyan T, et al. Adhesion molecules as prognostic markers in pancreatic adenocarcinoma. J Surg Oncol. 2007. 96:419–423.


33. Fais S, De Milito A, Lozupone F. The role of FAS to ezrin association in FAS-mediated apoptosis. Apoptosis. 2005. 10:941–947.


34. Krawetz R, MacKenzie MJ, Sun Q, Walton PA, Kelly GM. Galpha13 activation rescues moesin-depletion induced apoptosis in F9 teratocarcinoma cells. Exp Cell Res. 2006. 312:3224–3240.
35. Hayflick L, Moorhead PS. The serial cultivation of human diploid cell strains. Exp Cell Res. 1961. 25:585–621.


36. Weinman EJ, Steplock D, Donowitz M, Shenolikar S. NHERF associations with sodium-hydrogen exchanger isoform 3 (NHE3) and ezrin are essential for cAMP-mediated phosphorylation and inhibition of NHE3. Biochemistry. 2000. 39:6123–6129.
37. Shiue H, Musch MW, Wang Y, Chang EB, Turner JR. Akt2 phosphorylates ezrin to trigger NHE3 translocation and activation. J Biol Chem. 2005. 280:1688–1695.


38. Chorna-Ornan I, Tzarfaty V, Ankri-Eliahoo G, Joel-Almagor T, Meyer NE, Huber A, et al. Light-regulated interaction of Dmoesin with TRP and TRPL channels is required for maintenance of photoreceptors. J Cell Biol. 2005. 171:143–152.
39. Erwig LP, McPhilips KA, Wynes MW, Ivetic A, Ridley AJ, Henson PM. Differential regulation of phagosome maturation in macrophages and dendritic cells mediated by Rho GTPases and ezrin-radixin-moesin (ERM) proteins. Proc Natl Acad Sci U S A. 2006. 103:12825–12830.
40. Doulet N, Donnadieu E, Laran-Chich MP, Niedergang F, Nassif X, Couraud PO, et al. Neisseria meningitidis infection of human endothelial cells interferes with leukocyte transmigration by preventing the formation of endothelial docking structures. J Cell Biol. 2006. 173:627–637.


41. Elbashir SM, Harborth J, Lendeckel W, Yalcin A, Weber K, Tuschl T. Duplexes of 21-nucleotide RNAs mediate RNA interference in cultured mammalian cells. Nature. 2001. 411:494–498.
42. Brummelkamp TR, Bernards R, Agami R. A system for stable expression of short interfering RNAs in mammalian cells. Science. 2002. 296:550–553.
43. Dimri GP, Lee X, Basile G, Acosta M, Scott G, Roskelley C, et al. A biomarker that identifies senescent human cells in culture and in aging skin in vivo. Proc Natl Acad Sci U S A. 1995. 92:9363–9367.


44. van der Loo B, Fenton MJ, Erusalimsky JD. Cytochemical detection of a senescence-associated beta-galactosidase in endothelial and smooth muscle cells from human and rabbit blood vessels. Exp Cell Res. 1998. 241:309–315.


45. Severino J, Allen RG, Balin S, Balin A, Cristofalo VJ. Is beta-galactosidase staining a marker of senescence in vitro and in vivo? Exp Cell Res. 2000. 257:162–171.
47. Lou Z, Chen J. Cellular senescence and DNA repair. Exp Cell Res. 2006. 312:2641–2646.
48. Jacobs JJ, de Lange T. Significant role for p16INK4a in p53-independent telomere-directed senescence. Curr Biol. 2004. 14:2302–2308.


49. Iwasa H, Han J, Ishikawa F. Mitogen-activated protein kinase p38 defines the common senescence-signalling pathway. Genes Cells. 2003. 8:131–144.


50. Singh KK. Mitochondria damage checkpoint, aging, and cancer. Ann N Y Acad Sci. 2006. 1067:182–190.


51. Hara E, Smith R, Parry D, Tahara H, Stone S, Peters G. Regulation of p16CDKN2 expression and its implications for cell immortalization and senescence. Mol Cell Biol. 1996. 16:859–867.
52. Alcorta DA, Xiong Y, Phelps D, Hannon G, Beach D, Barrett JC. Involvement of the cyclin-dependent kinase inhibitor p16 (INK4a) in replicative senescence of normal human fibroblasts. Proc Natl Acad Sci U S A. 1996. 93:13742–13747.


53. Ressler S, Bartkova J, Niederegger H, Bartek J, Scharffetter-Kochanek K, Jansen-Durr P, et al. p16INK4A is a robust in vivo biomarker of cellular aging in human skin. Aging Cell. 2006. 5:379–389.
54. Verrills NM, Liem NL, Liaw TY, Hood BD, Lock RB, Kavallaris M. Proteomic analysis reveals a novel role for the actin cytoskeleton in vincristine resistant childhood leukemia--an in vivo study. Proteomics. 2006. 6:1681–1694.
55. Madan R, Brandwein-Gensler M, Schlecht NF, Elias K, Gorbovitsky E, Belbin TJ, et al. Differential tissue and subcellular expressionof ERM proteins in normal and malignant tissues: cytoplasmic ezrin expression has prognostic signficance for head and neck squamous cell carcinoma. Head Neck. 2006. 28:1018–1027.

