Abstract
Objective
To evaluate the relationship between CT dose and the performance of a computer-aided diagnosis (CAD) system, and to determine how best to minimize patient exposure to ionizing radiation while maintaining sufficient image quality for automated lung nodule detection, by the use of lung cancer screening CT.
Materials and Methods
Twenty-five asymptomatic volunteers participated in the study. Each volunteer underwent a low-dose CT scan without contrast enhancement (multidetector CT with 16 detector rows, 1.25 mm section thickness, 120 kVp, beam pitch 1.35, 0.6 second rotation time, with 1.25 mm thickness reconstruction at 1.25 mm intervals) using four different amperages 32, 16, 8, and 4 mAs. All series were analyzed using a commercially available CAD system for automatic lung nodule detection and the results were reviewed by a consensus reading by two radiologists. The McNemar test and Kappa analysis were used to compare differences in terms of the abilities to detect pulmonary nodules.
Results
A total of 78 non-calcified true nodules were visualized in the 25 study subjects. The sensitivities for nodule detection were as follows: 72% at 32 mAs, 64% at 16 mAs, 59% at 8 mAs, and 40% at 4 mAs. Although the overall nodule-detecting performance was best at 32 mAs, no significant difference in nodule detectability was observed between scans at 16 mAs or 8 mAs versus 32 mAs. However, scans performed at 4 mAs were significantly inferior to those performed at 32 mAs (p < 0.001).
Recently, low-dose CT has been used as a screening and early detection tool for lung cancer (1-6). Kaneco et al. (6) compared the use of low-dose spiral CT with radiography for the early detection of small peripheral lung cancers in a high-risk population, and found that the use of low-dose spiral CT is superior to the use of chest radiography. However, Nitta et al. (5) described many issues concerning lung cancer screening by CT, and concluded that issues such as economic viability, safety concerns (i.e., radiation dose), and the ability to accommodate widespread screening, remain to be resolved.
One of the major concerns of using CT for lung cancer screening is the burden imposed on radiologists, and this will continue to increase given the continued adoption of low-dose CT for lung cancer screening. Therefore, a suitable computer-aided detection (CAD) system is required to assist radiologists during the lung nodule detection process, and as a result, many researchers have advocated the usefulness of CAD systems for lung nodule detection (7-12).
Another major concern is that even low-dose helical CT exposes patients to more radiation than in chest radiography (5). Although low- to ultra-low-dose chest CT has been used, conventional 50 mA low-dose CT is normally used for lung cancer screening (3, 6, 13). Moreover, previous studies on low-dose or ultra-low-dose CT were performed without a CAD system (3, 5, 6, 13), and no comparative information is available in the English language clinical literature on low-dose and ultra-low-dose CT supported by a CAD system. In the present study, we assessed the rationale and feasibility of the use of ultra-low-dose helical CT using a CAD system. The purpose of our study was to evaluate the relationship between CT dose reduction and CAD system detection performance, and to determine how best to minimize patient exposure to ionizing radiation while maintaining a sufficient image quality for automated lung nodule detection during lung cancer screening by the use of CT.
The institutional review board approved this prospective study, and written informed consent was obtained from all 25 study subjects.
The study cohort was composed of 25 asymptomatic volunteers (13 men, 12 women) between the ages of 35 and 77 years (mean age, 62 years). Subjects were divided by body status into two groups. At first, body mass index (BMI) was calculated from subject height and weight. Subjects with a BMI of 25 or more were included in the obese group and subjects with a BMI of less than 25 were included into the normal or slender group. The overall mean BMI was 24.3 kg/m2 (range, 18.9-29.3 kg/m2). There were 11 patients with a BMI of 25 or more; 14 patients had a BMI of less than 25.
All CT examinations were performed using a Light Speed 16 Scanner (General Electric Medical Systems, Milwaukee, WI). Intravenous contrast medium was not injected into any subject. Volumetric helical CT scans were obtained through the thorax using four different tube currents 32, 16, 8 and 4 mAs per slice in each patient consecutively and without having the patients leave the scanner between scans. The entire scanning schedule took less than 5 minutes per patient. The scanning parameters used were 120 kVp, 16 × 1.25 mm detector thickness set, beam pitch 1.35 and a 0.6 second gantry rotation time. Transverse images were reconstructed using a 36-cm display field of view, a 1.25-mm section thickness, and at 1.25-mm intervals using a high spatial frequency (bone) reconstruction algorithm.
To determine the actual amount of radiation delivered at different tube currents, we measured the absorbed radiation dose. Radiation doses applied to lungs were obtained from direct thermoluminescence dosimeter measurements using an anthropomorphic Rando phantom (model RAN-110, Churchin Associates, Smithtown, NY), and lithium fluoride chips (TLD-100 [3.2 × 3.2 × 0.9 mm3], Thermo RMP, Solon, OH). Two lithium fluoride chips were placed in the center of the phantom lungs along its main axis, i.e., one in the right and in the left lungs. Measurements were performed four times at each of the four tube currents. Organ and tissue doses were assessed by calculating average absorbed doses.
Network transfer of CT data from a picture archiving and communication system (Centricity 2.0, General Electric Medical Systems, Milwaukee, WI) to a CAD workstation was realized using the DICOM protocol. Images were displayed and were processed using a commercially available software program (Extended Brilliance Workspace v 2.0, Philips Medical Systems, Cleveland, OH). Both mediastinal (window width, 400 Hounsfield units [HU]; window level, 20 HU) and lung (window width, 1,500 HU; window level, -700 HU) window images were visualized on workstation monitors.
Two radiologists (a radiology resident and a thoracic radiologist with 10 years experience) carefully reviewed four sets of chest CT scans for non-calcified true lung nodules between 3 and 20 mm in diameter for reference purposes. The numbers, sizes, and locations of the true nodules were recorded. Each of the CT scans was then reviewed using the CAD system, and details of the candidate lesions were recorded.
The two reviewers evaluated each CT scan and compared the CAD results, and decided by consensus whether the pulmonary nodules detected by CAD represented true or false nodules. The number of true positive and false positive nodules and their locations were documented.
The sensitivities and number of false- and true-positive nodules detected using CAD were computed for each current based on the radiologists' consensus opinions. However, because true-negative findings could not be recorded for individual nodules in the lungs, specificities and negative predictive values could not be calculated.
The McNemar test and Kappa analysis were used to compare differences in pulmonary nodule detectabilities in the same lungs at 4, 8, and 16 mAs versus 32 mAs. The Mann-Whitney test was used to compare the differences in pulmonary nodule detectabilities between different locations and different BMI groups. P values of < 0.05 were taken to indicate statistical significance.
Transverse CT scans acquired at the four tube currents are shown in Figure 1. The image quality expectedly decreased and noise increased as the tube currents were reduced. The radiation doses actually delivered at each of the 4 tube currents are summarized in Table 1, and in particular, radiation delivered to organs at 8 mAs was less than a quarter of that delivered at 32 mAs.
The total number of nodules detected, including true and false positive nodules, was 149 nodules at 32 mAs, 138 nodules at 16 mAs, 161 nodules at 8 mAs and 139 nodules at 4 mAs based on use of the CAD system. Twenty-three of the 25 patients had nodules seen in the CT scans. A total of 78 non-calcified true nodules (3.1 per person) were determined to be true nodules and were used as the reference standard. The numbers of pulmonary nodules and the sensitivities of each series of 4 tube currents are summarized in Table 2. Of these 78 nodules, the CAD system allowed the reviewers to identify 56 nodules (at 32 mAs), 50 nodules (at 16 mAs), 46 nodules (at 8 mAs) and 31 nodules (at 4 mAs), corresponding to sensitivities of 72%, 64%, 59%, and 40%, respectively. Sensitivity differences were not significant between 32 mAs and 16 mAs (p = 0.361, k = 0.3624) or between 32 mAs and 8 mAs (p = 0.123, k = 0.2882). Although images acquired at 16 and 8 mAs were less well resolved than those obtained at 32 mAs, and the overall nodule detection was best at 32 mAs, no statistically significant degradation in nodule detectability was found at 16 mAs or 8 mAs versus 32 mAs. However, the number of nodules detected were significantly different at 4 mAs than at 32 mAs (p < 0.0001, k = 0.2666) (Fig. 2). The average number of false-positive nodules at the individual current setting were 3.7 at 32 mAs, 3.5 at 16 mAs, 4.6 at 8 mAs, and 4.3 at 4 mAs on a per patient basis. No statistical differences were found for the average number of false-positive nodules at 16 mAs, 8 mAs, and 4 mAs compared to that for 32 mAs (p values were 0.685, 0.139 and 0.387, respectively, using the paired sample t-test).
With regard to the nodule locations, the CAD system better identified nodules in the lower lobes than in the upper or middle lobes at all tube currents (Table 3). In terms of nodule detection in the upper lobes, the sensitivity rates were 71% (20 of 28) at 32 mAs, 61% (17 of 28) at 16 mAs, 57% (16 of 28) at 8 mAs and 32% (9 of 28) at 4 mAs. In the lower lobes, the sensitivities were 82% (23 of 28) at 32 mAs, 64% (18 of 28) at 16 mAs, 57% (16 of 28) at 8 mAs and 50% (14 of 28) at 4 mAs. However, although the detectability of nodules in the lower lobes was better than in the upper lobes, the difference was not statistically significant (p = 0.222, Mann-Whitney test).
With regards to the BMI, the CAD system better identified nodules in slim patients than in obese patients at all tube currents (Table 4). For nodules in patients with a BMI of less than 25, the detection sensitivities were 78% (40 of 51) at 32 mAs, 65% (33 of 51) at 16 mAs, 65% (33 of 51) at 8 mAs, and 53% (27 of 51) at 4 mAs. In patients with a BMI over 25, detectabilities were 59% (16 of 27) at 32 mAs, 63% (17 of 27) at 16 mAs, 48% (13 of 27) at 8 mAs, and 15% (4 of 27) at 4 mAs. Moreover, the lung nodule detection sensitivity was significantly better in patients with a BMI of less than 25 (p = 0.001, Mann-Whitney test).
The goal of lung cancer screening CT is the detection of small cancers, presumably when they are at a biologically early stage. The 5-year survival rate after resection of stage IA non-small cell lung cancer is 62-82% (14), and the outcomes of patients who decline treatment for stage I cancer is dismal (15). This is why many investigators in the medical community are hopeful that CT screening will provide a means of detecting early stage lung cancer. During the past few years, low-dose CT has been accepted to be a highly sensitive modality to use for detecting small pulmonary nodules (6, 16, 17). Kaneko et al. (6) described 15 cancers that were found during 3,457 routine screening CT examinations; only four of these cancers were evident by chest radiography, and 14 were at stage I. Swensen et al. (16) reported 40 cases of lung cancers in 1,049 CT examinations, and found that CT alone depicted 36 cases, whereas a sputum cytological examination alone detected only two cancers. Henschke and colleagues (17) evaluated the low-dose CT scans of 1,000 asymptomatic individuals aged 60 years or more, with a history of at least 10 pack-years of cigarette smoking, and compared findings with those of chest radiography. It was found that low-dose CT markedly better detected small non-calcified nodules, and better-detected early stage lung cancer. Moreover, low-dose CT detected three times as many non-calcified nodules, four times as many malignancies, and six times as many stage I malignancies than chest radiography.
However, the radiation risk posed by low dose CT is not negligible. Calculations made by Brenner (22) revealed that if 50% of all current and former smokers aged 50-75 years in the U.S. received annual CT screening, lung cancers associated with radiation due to screening would increase in patients that received the routine screening chest CT scan by 2%.
The relationship between radiation exposure and biological risk to patients is determined by extrapolation, based on changes observed after exposure to higher levels of radiation (18). In addition, patient age and sex should be considered when analyzing risk; for example, the delivery of 1 rad (10 mGy) to the breast of a woman younger than 35 years old is estimated to increase the risk of breast cancer by approximately 14% over the spontaneous rate in the general population (19).
Tube current reductions cause proportionate reductions in the delivered radiation dose, as the dosage is linearly related to amperage at a fixed tube voltage (13, 20, 21). The radiation dose delivered during routine chest CT scans using a sixteen-channel multi-detector CT (120 kVp, 150 mAs, 20-mm beam collimation, and 1.67 beam pitch) at our institution is about 14 mGy, and the radiation dose of conventional low-dose CT (120 kVp, 33 mAs, 20-mm collimation, and 1.35 beam pitch) is 6.1 mGy. In contrast, the radiation dose delivered by ultra-low-dose CT at 8 mAs (120 kVp, 20-mm collimation, and 1.35 beam pitch) is 1.5 mGy; a quarter of the dose delivered by conventional low-dose CT.
Some researchers (13, 23) have concluded that image quality and diagnostic accuracy do not deteriorate when tube currents are reduced within a certain range. Therefore, in order to reduce the radiation dose and radiation associated risks, some investigators have used ultra-low-dose CT protocols. Rusinek et al. (23) compared 200 CT image panels acquired at 20 mAs and 200 mAs using a four-point confidence scale based on lesion sizes, locations, and the relationship between the blood vessels and pulmonary nodules. In this previous study, six observers compared images acquired at these two currents (doses), and of 864 positive panels, 259 (60%) of 432 low-dose panels and 272 (63%) of 432 conventional panels were correctly interpreted (p = 0.259). Naidich et al. (13) compared CT scans obtained at 140 mAs and 10 mAs with all other parameters held constant, and found that at all thorax levels, visualizations of parenchymal structures were unaffected by reducing tube currents. However, these studies used higher tube currents than those used in the present study.
Other investigators have concluded that ultra-low dose CT produces unacceptable image qualities. Mayo et al. (24) found that reducing chest CT radiation dose to 40 mA degraded image quality and reduced reader diagnostic accuracy. In the present study, axial images obtained at 16, 8, and 4 mAs also showed increasing noise and poorer image clarity.
We were curious as to whether the CAD system would enable us to avoid the limitations associated with low photon noise and low image contrast caused by reducing the tube current. Theoretically, quantum noise increases inversely in proportion to the square root of the amperage. However, because the lungs are aerated, they generate more contrast than solid organs, and the detection of pathological changes in the lung are less dependent on image noise than for those in solid organs (3).
In the present study, we found no difference in nodule detection rates using CAD between 32, 16, and 8 mAs. Therefore, we assert that 8 mAs is the lowest setting that can be employed without losing pulmonary nodule detectability using CAD in normal or slim patients. However, this limitation could be managed using automatic dose modulation based on an individual BMI.
A limitation of this study is that no histological data on lung nodules was incorporated into the present study. We found the nodules in 23 of 25 patients, and this proportion is unusually higher than that of normal population (35%) reported previously by Chong et al. (1). Furthermore, the average of the number of true nodules (3.1 per person) from 23 patients that had nodules was more than the value determined (1.8 per person) by Chong et al. We assume this finding resulted, as the volunteers that were included in our study were not truly a healthy population. Of course, the subjects were asymptomatic, and most of the candidates that wanted to be included in this study have a history of previous disease such as tuberculosis or breast cancer.
In conclusion, reducing the radiation dose lowers lung nodule detectability as determined by the use of by CAD. However, relatively low dose scans were found to be acceptable using CAD, and in particular, to cause no significant reduction in nodule detectability in comparison with usual low-dose CT.
Figures and Tables
Fig. 1
Transverse CT scans were acquired at tube currents of 32 (A), 16 (B), 8 (C), and 4 (D) mAs. Note that image quality was reduced and noise was increased as tube currents were decreased.
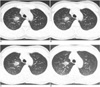
Fig. 2
Five-mm nodule in right lower lobe superior segment, which was detected at 32 (A), 16 and 8 mAs/slice (not shown in this figure), but not at 4 mAs/slice (C) on the CAD system. Note that volume-rendering image obtained at 32 mAs (B) is sharper than that obtained at 4 mAs (D).
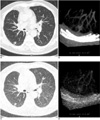
References
1. Chong S, Lee KS, Chung MJ, Kim TS, Kim H, Kwon OJ, et al. Lung cancer screening with low-dose helical CT in Korea: experiences at the Samsung Medical Center. J Korean Med Sci. 2005. 20:402–408.
2. Gergely I, Neumann C, Reiger F, Dorffner R. Lung nodule detection with ultra-low-dose CT in routine follow-up of cancer patients. Rofo. 2005. 177:1077–1083.
3. Karabulut N, Toru M, Gelebek V, Gulsun M, Ariyurek OM. Comparison of low-dose and standard-dose helical CT in the evaluation of pulmonary nodules. Eur Radiol. 2002. 12:2764–2769.
4. Nitta N, Takahashi M, Murata K, Morita R. Ultra low-dose helical CT of the chest: evaluation in clinical cases. Radiat Med. 1999. 17:1–7.
5. Nitta N, Takahashi M, Murata K, Morita R. Ultra low-dose helical CT of the chest. AJR Am J Roentgenol. 1998. 171:383–385.
6. Kaneko M, Eguchi K, Ohmatsu H, Kakinuma R, Naruke T, Suemasu K, et al. Peripheral lung cancer: screening and detection with low-dose spiral CT versus radiography. Radiology. 1996. 201:798–802.
7. Marten K, Engelke C, Seyfarth T, Grillhosl A, Obenauer S, Rummeny EJ. Computer-aided detection of pulmonary nodules: influence of nodule characteristics on detection performance. Clin Radiol. 2005. 60:196–206.
8. Armato SG 3rd, Roy AS, Macmahon H, Li F, Doi K, Sone S, et al. Evaluation of automated lung nodule detection on low-dose computed tomography scans from a lung cancer screening program(1). Acad Radiol. 2005. 12:337–346.
9. Lee JW, Goo JM, Lee HJ, Kim JH, Kim S, Kim YT. The potential contribution of a computer-aided detection system for lung nodule detection in multidetector row computed tomography. Invest Radiol. 2004. 39:649–655.
10. Awai K, Murao K, Ozawa A, Komi M, Hayakawa H, Hori S, et al. Pulmonary nodules at chest CT: effect of computer-aided diagnosis on radiologists' detection performance. Radiology. 2004. 230:347–352.
11. Arimura H, Katsuragawa S, Suzuki K, Li F, Shiraishi J, Sone S, et al. Computerized scheme for automated detection of lung nodules in low-dose computed tomography images for lung cancer screening. Acad Radiol. 2004. 11:617–629.
12. Goo JM, Lee JW, Lee HJ, Kim S, Kim JH, Im JG. Automated lung nodule detection at low-dose CT: preliminary experience. Korean J Radiol. 2003. 4:211–216.
13. Naidich DP, Marshall CH, Gribbin C, Arams RS, McCauley DI. Low-dose CT of the lungs: preliminary observations. Radiology. 1990. 175:729–731.
14. Flehinger BJ, Melamed MR. Current status of screening for lung cancer. Chest Surg Clin N Am. 1994. 4:1–15.
15. Flehinger BJ, Kimmel M, Melamed MR. The effect of surgical treatment on survival from early lung cancer. Implications for screening. Chest. 1992. 101:1013–1018.
16. Swensen SJ, Jett JR, Hartman TE, Midthun DE, Sloan JA, Sykes AM, et al. Lung cancer screening with CT: Mayo Clinic experience. Radiology. 2003. 226:756–761.
17. Henschke CI. Early lung cancer action project: overall design and findings from baseline screening. Cancer. 2000. 89:2474–2482.
18. Nickoloff EL, Alderson PO. Radiation exposures to patients from CT: reality, public perception, and policy. AJR Am J Roentgenol. 2001. 177:285–287.
19. Remy-Jardin M, Remy J. Spiral CT angiography of the pulmonary circulation. Radiology. 1999. 212:615–636.
20. Zhu X, Yu J, Huang Z. Low-dose chest CT: optimizing radiation protection for patients. AJR Am J Roentgenol. 2004. 183:809–816.
21. Jung KJ, Lee KS, Kim SY, Kim TS, Pyeun YS, Lee JY. Low-dose, volumetric helical CT: image quality, radiation dose, and usefulness for evaluation of bronchiectasis. Invest Radiol. 2000. 35:557–563.
22. Brenner DJ. Radiation risks potentially associated with low-dose CT screening of adult smokers for lung cancer. Radiology. 2004. 231:440–445.
23. Rusinek H, Naidich DP, McGuinness G, Leitman BS, McCauley DI, Krinsky GA, et al. Pulmonary nodule detection: low-dose versus conventional CT. Radiology. 1998. 209:243–249.
24. Mayo JR, Kim KI, MacDonald SL, Johkoh T, Kavanagh P, Coxson HO, et al. Reduced radiation dose helical chest CT: effect on reader evaluation of structures and lung findings. Radiology. 2004. 232:749–756.