Abstract
High-intensity focused ultrasound therapy is a novel, emerging, therapeutic modality that uses ultrasound waves, propagated through tissue media, as carriers of energy. This completely non-invasive technology has great potential for tumor ablation as well as hemostasis, thrombolysis and targeted drug/gene delivery. However, the application of this technology still has many drawbacks. It is expected that current obstacles to implementation will be resolved in the near future. In this review, we provide an overview of high-intensity focused ultrasound therapy from the basic physics to recent clinical studies with an interventional radiologist's perspective for the purpose of improving the general understanding of this cutting-edge technology as well as speculating on future developments.
Focusing the sunrays onto a small spot with a magnifying glass to start a fire is a childhood experiment that many of us tried. High-intensity focused ultrasound (HIFU) therapy is a technology with similar principles using ultrasound (US) instead of sunrays. HIFU therapy can transport energy in the form of US waves through a media of intervening tissues to specific target points of body organs, and hence, increase the temperature or bring about other biological interactions in an absolutely non-invasive manner. No significant negative biological effects on the intervening tissue occurs as long as that the ultrasonic energy is appropriately located and focused. Because of its non-invasive nature, this technology has attracted the attention of clinicians, investigators and companies from around the world as an innovative, interventional tool that might provide virtually complication-free therapy.
The use of US for therapy predates its application in diagnosis. The biological effects of HIFU were recognized in 1927 (1). Since the 1930s, unfocused, usually low-intensity US has been adopted for physiotherapy (2). In 1942, Lynn et al. (3) demonstrated that highly localized biological effects could be produced by focusing US. In the 1950s, focused US was employed for brain therapy through a soft tissue window by Drs. Fry for the first time (4, 5), and then, clinical application was attempted for Parkinson's disease (6). However, this clinical application was overshadowed by the development of L-dopa, which was considered very successful at the time. After a long period of relative inactivity, technological advances within the past 10 or more years have caused a resurgence of this technology in clinical medicine. The first report on the clinical use of HIFU for prostate cancer was published in 1994 (7), which was followed by many additional clinical studies on its use on a variety of body organs.
Although most clinical studies on HIFU therapy have dealt only with thermal ablations (focused US surgery; FUS) (discussed later), the range of its potential applications in medical science appears to be much wider when numerous on-going investigations on hemostasis, thrombolysis and targeted drug/gene-delivery systems are considered.
In this review, we provide information encompassing the basic physics of sound required for understanding this therapy, biological interactions of US, the mechanisms of action of HIFU therapy, clinical HIFU devices, methods for guiding and monitoring therapy, and the results of clinical studies from the viewpoint of interventional radiologists. Our goal is to improve the general understanding of the status of technological development of HIFU therapy as well as speculate on the future direction of this novel technology.
Sound is defined as a disturbance of mechanical energy that propagates through a medium in the form of waves. As this definition implies, sound can transport energy from its source to another area as long as a medium is present. US is a form of sound that has a higher frequency (> 20,000 Hz) than the human ear can detect (20-20,000 Hz; audible ranges). The important parameters of sound are summarized in Figure 1.
While other minimally invasive therapies such as radiofrequency ablation or microwave ablation use an electrode or antenna to deliver electromagnetic waves, HIFU therapy makes use of US waves as carriers of energy, which is propagated through human tissues. US has been shown to have no detrimental effect on the human body within the diagnostic ranges used (8, 9). However, it must be noted that US waves carry energy that causes biological reactions in various ways (discussed later) although these are usually minimal. The main challenge of this technique is to maximize energy-accumulation at the target area in order to induce significant biological reactions without causing harm to the intervening tissues such as the skin and the tissues surrounding the target area. HIFU therapy has adopted two strategies to resolve this problem. It uses high energy US waves generated from numerous sources and focuses them to a small spot (Fig. 2).
Sonic intensity (SI) can be defined as a time-average rate of sonic energy-flow through a unit area (SI unit: W/cm2). The sonic intensity is proportional to sonic pressure square and has a positive correlation with the power and energy of sound (10). This implies that the higher the sonic pressure or intensity is, the larger the energy accumulation at the target area is (Fig. 3). Sonic intensity varies with space and time, and it is usually expressed as peak or average intensity, and both quantities can refer to either a spatial or temporal dimension (e.g. ISP = spatial peak intensity, ISATA = spatial average, temporal average intensity) (11, 12). In general, tissue-heating by US absorption is best predicted by the average intensity and the activity of acoustic cavitation by peak intensity (12). High-intensity US generally refers to US with an intensity (ISATA) higher than 5 W/cm2. This type of US can transfer enough energy to cause coagulation necrosis of tissue and is usually used for ultrasonic surgery. By contrast, low-intensity US (ISATA = 0.125-3 W/cm2) causes non-destructive heating, therefore, it stimulates or accelerates normal physiological responses to an injury. This range of US is usually used for physiotherapy (13).
The various methods of focusing US waves have been another important issue. The simplest and cheapest (often most accurate) method may be a shelf-focusing, for instance, a spherically curved US source (transducer). An US transducer constructed according to this method, has a beam focus fixed at the position determined from the geometrical specifications of the transducer. To compensate for its lack of versatility, a flat US transducer with an interchangeable acoustic lens system was devised. The acoustic lens enables variation of focusing properties such as focal length and focal geometry. However, a drawback of the lens system is that US waves undergo sonic attenuation due to absorption by the lens (14). Recently, a phased-array US transducer technique was adopted for HIFU therapy. By sending temporally different sets of electronic signals to each specific transducer component, this technique enables beam-steering and focusing, which can move a focal spot in virtually any direction within physically allowed ranges. This system is not only more versatile than other systems but also highly efficient without any sonic attenuation (15) (Fig. 4).
US beyond the diagnostic ranges can bring about various kinds of reactions when insonated into biological tissue. The resulting effects include thermal, mechanical, chemical and optical reactions. Mechanical effects, more specifically, may consist of acoustic cavitation, radiation force, shear stress, and acoustic streaming/microstreaming. Among them, the thermal effect and acoustic cavitation are the most significant, and their mechanisms of action have been relatively well-understood (16).
The thermal effect is caused by the absorption of US into biological tissue. US waves cause vibration or rotation of molecules or part of macromolecules in the tissue, and this movement results in frictional heat. Depending on the temperature and the duration of contact, the tissue may become more susceptible to chemotherapy or radiotherapy (> 43℃, 1 hour) or alternatively, protein denaturation may occur (coagulation necrosis) (56℃, 1 sec) (12, 16, 17) as shown in Figure 2. Excluding the effects of thermal transfer, the temperature-elevation of biological tissue by US (plane wave) absorption is theoretically linearly-proportional to sonic intensity in the following manner (∂T/∂t = 2αI/ρCP = 0.014 I; T = temperature [℃], t = time [sec],α= absorption coefficient [≈0.03 Np/cm in tissue-like medium at 1 MHz], I = sonic intensity, ρ= density [≈1 g/cm3 in tissue-like medium], Cp = specific heat [≈4.2 J/g℃ in tissue-like medium]) (18, 19). Because of this linearity and predictability, a thermal effect was traditionally preferred to a mechanical effect in the medical applications of FUS.
Acoustic cavitation, defined as the formation and activity of a gas- or vapor-filled cavity (bubble) in a medium exposed to an US field, plays a major role in the mechanical effects and minor roles in the chemical and the optical effects of US technology. If an US wave, more intense than a specific threshold, is insonated into biological tissue, negative pressure representing the rarefaction of an US wave, may be large enough to draw gas out of the tissue solution to form a bubble. It is easy to understand the underlying mechanism if this is compared to the numerous bubbles formed by vigorous rotation of a motorboat screw. This bubble either repeats radial oscillations in a resonant size with the insonated frequency (stable cavitation; non-inertial cavitation) or oscillates in a similar manner expanding gradually above its resonant size due to net influxes of vapor into the bubble (rectified diffusion), and finally disintegrates by a violent and asymmetrical collapse (unstable cavitation; inertial cavitation) (8, 16) (Table 1). Acoustic cavitation, particularly inertial cavitation, can cause a significant degree of mechanical and thermal effects as well as chemical and optical effects. The thermal effect caused by acoustic cavitation is larger than that caused by US absorption alone. Mechanical and thermal effects by acoustic cavitation are generally known to be complex, unpredictable, and, sometimes, detrimental. The threshold of acoustic cavitation depends on (negative) pressure amplitude and frequency of the sound and the tissue where cavitation occurs (8, 16, 20).
Radiation force is a force exerted at an interface between two media or inhomogeneity in a medium due to the passage of US waves. An acoustic field in fluid may set up acoustic streaming; the transfer of momentum to liquid, by the absorption of energy from an acoustic field, causes acoustic streaming. The fluid velocity caused by acoustic streaming is spatially non-uniform thereby generating a velocity gradient in the field. This gradient causes shear stress. Acoustic streaming caused by an oscillating bubble in a sound field immediately surrounding the bubbles is specifically referred to as acoustic microstreaming. Shear stress formed by microstreaming is an important mechanism underlying many biological reactions (8, 16).
Focused ultrasound surgery is used for local ablation therapy of various types of tumors using HIFU (ISA = 100-10,000 W/cm2). The two main mechanisms involved in FUS are thermal effects by US absorption and mechanical effects involving thermal effects in part, induced by acoustic cavitation. The thermal effect by absorption has been traditionally employed because it is relatively accurately predictable and thus easy to control. This enables the therapy to be safe even though thermal ablation by the conventional method of FUS generally requires a long surgical time for clinical practice. the effects of cavitation have proven to have potential in improving the efficiency of the therapy by enlarging the ablation size and subsequently reducing the procedure-time for ablation (21). However, these advantages could be accompanied by a longer cooling time and a relatively high risk of complication.
The shape of a classical thermal lesion resembles a cigar, paralleling the direction of the US propagation, measuring about 1.5-2 mm in width and about 1.5-2 cm in length when produced by a typical clinical 1.5 MHz HIFU field as shown in Figure 5 (12, 22). This single thermal lesion is extremely small in comparison to the sizes of common clinical tumors. The individual thermal lesions are stacked up closely without leaving intervening viable tissue to form a sufficient ablation zone to cover the tumor itself as well as the safety margin. The tissue-homogeneity influences the shapes of the thermal lesion while the tissue-perfusion may affect its size. The frequency of US is adjusted to optimize surgical conditions, keeping sonic attenuation low (advantage of low frequency) as well as making energy focused sharply enough (advantage of high frequency) (22).
The histological changes made by FUS have been investigated. Thermal damage after US absorption has been described as an "island and moat" in which the "island" represents an area of complete coagulation necrosis and complete destruction of the tumor-supplying vessels whereas the "moat" refers to the surrounding rim-like area that is 6-10 cells-thick and composed of glycogen-poor cells (-2 hours) that usually die within 48 hours. Later, granulation tissue, fibroblast infiltrates and finally retraction/scar formation occurs (22, 23). The changes that occur because of acoustic cavitation are both coagulation necrosis and mechanical tearing. Mechanical tearing, which is attributed to tissue boiling as well as the mechanical effects of acoustic cavitation, manifests as holes or implosion cysts upon microscopic examination (24).
Application of HIFU therapy to hemostasis was primarily initiated in an attempt to control battlefield injuries on the spot. High-intensity US (ISA = 500-3,000 W/cm2) is usually adopted for hemostasis. Many studies on animal models have been successful for both solid organ and vascular injuries (25).
The thermal effect has a major role in hemostasis. The proposed mechanisms of its action are as follows. Structural deformation of the parenchyma of a solid organ due to high temperature induces a collapse of small vessels and sinusoids or sinusoid-like structures. Heat also causes coagulation of the adventitia of vessels, and subsequently, fibrin-plug formation. The mechanical effect of acoustic cavitation also appears to play a minor role in hemostasis. Microstreaming induces very fine structural disruption of the parenchyma to form a tissue homogenate that acts as a seal and induces the release of coagulation factors (25). No statistically significant hemolysis or changes in the number of white blood cells and platelets have been observed when blood is exposed to HIFU with intensities up to 2000 W/cm2 (26).
US can play a significant role in thrombolysis. US with/without a thrombolytic agent has been shown to be effective in enhancing thrombolysis. Thrombolysis is achieved with low intensity US (ISA = 0.5-1 W/cm2) and is known to be associated with non-thermal mechanisms (27, 28). Microstreaming by acoustic cavitation produces a strong mechanical force around the cell membranes that causes the pores or channels to open. This increases the bioavailability of thrombolytic agents on the surface of a thrombus. The radiation force of the US itself could push the drug to the lesion ("push effect"). The direct mechanical effect with/without microstreaming could cause alterations to the fibrin mesh. These effects, described above, are believed to work synergistically to cause thrombolysis (29).
There are two methods of delivering US to thrombosed vessels. One is an extracorporeal approach. This is noninvasive, but requires higher US energy for compensating attenuation through an intervening tissue; in addition, it may have the potential risks of complications and treatment-failure due to the intervening tissues. Clinical trials using the extracorporeal low frequency US (as in transcranial Doppler US) for brain ischemia with the assistance of a tissue plasminogen activator have turned out to be successful (30). The other method is via a miniaturized transducer, at the tip of an arterial catheter, from which a thrombolytic drug is released. This system is minimally invasive and commercially available (EKOS® EndoWave® Pheripheral Infusion System, EKOS® NeuroWave® Catheter; EKOS® Co., Bothell, WA).
Although US-assisted thrombolysis was discussed separately, it is a specific type of targeted drug-delivery system. US-assisted targeted drug-delivery and gene therapy share a common mechanism where microbubbles play a critical role. Microbubbles are the vehicles used for drug or plasmid DNA (deoxyribonucleic acid)-delivery either in an encapsulated or an attached form. When these specially manipulated microbubbles pass through blood vessels, US (ISA = 0.5-1 W/cm2) is insonated selectively to the target area to which the therapeutic agents should be delivered. The US waves rupture the microbubbles, from which drugs/genes are released. Furthermore the microbubbles act as cavitation nuclei, thereby allowing the acoustic cavitation to take place more easily and on a greater scale. Violent microstreaming formed by the rupture of the microbubbles enhances the uptake of drugs/genes into the cells by sonoporation (a transient alteration of cell membrane structures due to mechanical force of US) as well as the "push effect" (Fig. 6) (29, 31, 32). One of the problems of conventional gene therapy, using viral vectors for gene delivery, is the unwanted adverse effects of systemic immune responses. US-enhanced gene therapy can prevent this problem. By targeting delivery only to the diseased area, it can increase the concentration of therapeutic agents at a focused area of disease and lower the probability of systemic complications (32).
There are two specialized forms of US-enhanced drug delivery. Sonopheresis is one method, which involves the US-enhanced penetration of pharmacologically-active agents through the skin or other anatomical barriers. It is mediated by acoustic cavitation and microstreaming, which renders the stratum corneum of the skin temporarily permeable. This method has had a great clinical impact on the dermal administration of insulin to diabetics. The second method is sonodynamic therapy, which makes use of US to activate photosensitive or sonosensitive drugs. The exact mechanism is unknown, but the sonoluminescence phenomenon resulting from acoustic cavitation is believed to be involved (12, 29).
Radiologists who are accustomed to diagnostic US within the frequency range of 3-12 MHz may harbor the misconception that US cannot pass through bony structures. Bone significantly absorbs and reflects US waves because it has an attenuation coefficient, therefore, it has an acoustic impedance much higher than those of the surrounding soft tissues. Some percentages of incident US, even if very small, may be transmitted through bone if its wavelength is larger than the thickness of bone, for instance, frequencies lower than 1 MHz in the case of the skull. This results in very poor efficiency of energy transfer and excessive heating of the skull in transcranial US therapy. Another problem of the transcranial US therapy is the severe aberration of US waves. This is due to an irregularity of the skull-thickness and a high speed of sound in the bone resulting in the defocusing of US beams. To overcome this low-efficiency problem, a transducer with a large number of high-energy sources is currently being used. To lower the skull temperature, investigators have adopted an external cooling system that circulates chilled water around the scalp. The active area is maximized by adopting a hemispheric design, referred to as a piezoelectric component arrangement, to distribute the heat as widely as possible. To minimize the defocusing problem, a computerized multi-channel phased-array transducer has been devised. Directions of the individual beams from the transducer are controlled by a computer-calculations based on CT-driven data of the skull thickness for each corresponding area to focus the US beams to a small sharply-margined area (15, 33) (Fig. 7). A 512-channel transducer and driving system have been developed and tested for the normal brain in vivo (34).
Focused US has also been shown to have the ability to induce selective opening of the blood brain barrier (BBB) without damaging normal neuronal tissue (35). This enables US-enhanced drug-delivery to specific areas of the diseased brain. Each application or combination of applications has had significant clinical implications because the conventional therapies including surgery have very limited roles in this field.
Intravenous microbubble agent injection during therapy enhances the effects of many different therapeutic responses where acoustic cavitation is known to be involved. The microbubbles injected act as cavitation nuclei, playing a role in seeding for cavitation and lowering the threshold of acoustic cavitation. This eventually increases the activity of acoustic cavitation (11, 12).
In FUS, microbubbles effectively absorb ultrasonic energy, resulting in a further enhancement of the tissue temperature and, under the same settings, a shortening of the sonication time required for treating the same sized tumors. These have been studied in in-vivo animal models (36-38). In cases using this technique clinically, the operator must be careful of complications caused by an excessive ablation. US-induced thrombolysis proved in animal models (39) as well as in stroke patients clinically (40) makes use of microbubble administration, which improves the effect of thrombolytic agents. Microbubbles can also enhance HIFU-induced hemostasis. They are effective in causing vascular thrombosis with a subtherapeutic dose of a thrombogenic agent in sclerotherapy (41) and can increase the efficiency of US-induced hemostasis in solid organ injury (42). As noted previously, the role of microbubble agents employed in targeted drug/gene-delivery are two-fold: as a vehicle for therapeutic agents and a cause of sonoporation, associated with the increased activity of acoustic cavitation (29, 31). Transcranial FUS of the brain and opening of the BBB are reported to be enhanced by the simultaneous administration of microbubbles in animal experiments (43, 44).
Since the 1990s, several commercial companies have developed different forms of clinical HIFU devices, which are now in the initial stages of their clinical applications. Generally, the devices are divided into transrectal and extracorporeal types according to their energy-delivery routes, and are also classified into US- and MR (magnetic resonance)-assisted devices according to their guiding and monitoring methods. Transrectal devices are exclusively used for the treatment of prostate pathology. There are two widely used clinical devices manufactured by companies in France (Ablatherm® HIFU system; EDAP, Vaulx-en-Velin) and the United States (Sonablate® 500 system; Focus Surgery, Inc., Indianapolis, IN). Both systems are guided and monitored by US imaging modalities.
Extracorporeal devices are relatively more versatile in application than transrectal. They can be used for benign or malignant pathology of the uterus, breast, liver, kidney, pancreas, thyroid, testis, extremities, and other organs where US can be delivered through an external surface of the human body. Clinically available extracorporeal HIFU devices have been developed by several companies in China (HAIFU System; Chongqing HIFU Technology, Co., Ltd., Chongqing, HIFU Tumor Therapy System; China Medical Technologies, Inc., Beijing, CZ901 HIFU System; Mianyang Sonic Electronic, Sichuan) and Israel (Exablate® 2000; InSightec, Haifa). Devices from China are US-assisted and those from Israel are MR-assisted. All Chinese devices utilize one or two single-element therapeutic transducers with an imaging transducer incorporated in their center. The transducers are spherically-curved so as to focus US waves to their geometrical focus and the area is mechanically manipulated in order to aim the US waves to the target spot. It has to be noted that the geometrical focus does not always coincide with the real beam focus at which the US intensity has its maximum. On the other hand, the MR-assisted device from Israel uses a phased-array transducer with approximately 200 elements that enables electronic manipulation of a focal zone within specific ranges. This range of focusing is complemented by the piezoelectric servo-motor system that also enables mechanical manipulation of the transducer.
The hyperechoic changes on B-mode US images do not actually depict a temperature elevation but reflects either the activity of acoustic cavitation or tissue boiling (45, 46). Therefore, there might be a possible mismatch in the locations between the hyperechoic changes and the real coagulation necrosis. In order to monitor the HIFU lesion directly and accurately, research on US thermometry are underway. The techniques employed may include changes in the speed of sound with temperatures of a medium (47) and US tissue elasticity imaging technique (48). Guiding and monitoring by US are relatively economical, completely real-time and can simulate HIFU beam propagation precisely because diagnostic and therapeutic US waves share a common pathway. However, the drawbacks include a relatively poor tissue-contrast, a limited field of view and a progressive deterioration of image quality as the treatment continues (17).
In contrast to US images, MR imaging modality provides excellent tissue-contrast and is not limited in terms of the field of view. MR can quantify changes in temperature and thermal dose (calculated value of equivalent time at a reference temperature of 43℃) of the treated tissue directly. MR thermometry makes use of the phenomenon of temperature sensitivity of the water proton resonance frequency (PRF) shift (49). A shift in the PRF is linearly related to temperature and can be mapped rapidly with standard MR imaging sequences using phase differences. However, the conventional MR thermometry is insensitive to temperature changes in fat and is susceptible to motion artifacts including tissue-swelling due to the need for image subtraction (15).
The clinical uses of HIFU therapy have been increasingly concentrated on treating tumors. The accessibility of US beams to the target organ is the most important determinant of whether or not HIFU therapy can be applied.
Prostate cancer has the longest history of clinical use of HIFU, therefore, many clinical studies have been performed on it. All of the clinical studies have been carried out with transrectal US-assisted equipment. FUS for early-stage, localized prostate cancer has been comparable to surgery in terms of local control, disease-free survival, and complication rates. The cumulative 5-year disease-free survival rates range from 68-78% (50-52). However, prospective randomized controlled trials have not been performed to date. FUS has also proven to be effective in the control of recurrent prostate cancer after an external beam radiation therapy (53).
The uterus provides a good target for FUS treatment because it is static and located close to the abdominal wall. Most clinical studies on uterine leiomyoma have been done with MR-assisted HIFU devices. It is the only disease entity that is approved by the FDA for treatment using this device at the time of writing this manuscript. FUS has been shown to be effective in controlling symptomatic uterine leiomyomas. Targeted symptom reduction rates have been reported to be 71% at six months and 51% at 12 months (54). However, the volume reduction rate of the tumors was not satisfactory (13.5% at 6 months) (55). Neoadjuvant use of GnRH (gonadotropin releasing hormone) turned out to improve both the symptom control rate (83% at 6 months, 89% at 12 months) and the volume reduction rate (21% at 6 months, 37% at 12 months) (56).
The breast is also a superficial and static organ. However, because of the difficulties in treating the axillary lymph nodes, the clinical application of HIFU therapy for breast cancer has been limited. The feasibility as a first line therapy has not been studied to date; only its local control rate has been evaluated (the local tumor progression rate 9.1%) (57, 58).
The liver, especially the right lobe, is not a suitable organ for the application of FUS because of the large respiratory excursions and the sonic shadowing caused by the ribs. Therefore, most clinical studies have been carried out on palliative applications rather than for curative purposes (59-61). FUS has proven to be effective in lengthening the survival of patients with advanced hepatocellular carcinomas in combination with transcatheter arterial chemoembolization (61). Liver cancer is a great prey of interventional oncologists. In order to overcome these problems, techniques utilizing FUS, which forms an excellent noninvasive weapon, are being investigated by researchers and manufacturers.
Pancreatic cancer is also a promising field for the palliative application of FUS. In one study, 100% of the patients experienced resolution of back-pain after the treatment (62). The effects of FUS on primary and metastatic renal cancers (63), malignant bone tumors (64), soft tissue sarcomas (64), testicular tumors (65), and brain tumors (66) also have been evaluated and most have found application for palliative purposes useful.
Major differences of HIFU therapy from other interventional therapeutic modalities are its complete non-invasiveness and sharp, tailorable treatment margins, which may lead to treatments with very low complication rates. However, several complications have been known to occur after HIFU therapy. These are mostly due to high-energy US waves reflected on gas or bony structures (54, 67). Skin-burn can be caused by poor acoustic coupling between the skin and the therapeutic window (e.g. poor shaving) or a previous operation scar. In cases of liver treatment, reflected US waves on ribs can induce overlying soft tissue damage including the skin. Gas-containing bowel loops act in the same manner and can cause thermal injury of the bowel wall. Sciatic nerve injury was also reported after HIFU therapy for uterine leimyoma. This complication is deemed to be caused either directly by high-energy US waves that pass the focal therapeutic zone or indirectly by elevated temperatures of the pelvic bone. If the focal zone is located superficially as in case of breast cancer, direct thermal injury of overlying skin can occur (58). Likewise, internal organs just anterior or posterior to the focal zone could be injured.
In addition to these complications, HIFU therapy at the time of writing this manuscript, has displayed several other limitations, which are hampering the effective use of this modality in clinical practice. These include a long procedure time, difficulty in targeting and monitoring moving organs, sonic shadowing by bones or gas in bowels, and the relatively high cost of this technique in relation to its effectiveness and limitations. However, recent technological advances are expected to resolve these problems. One example is the new MR-assisted HIFU device under development, which adopts the technique of an automatic on-line, spatiotemporal temperature control using a multispiral trajectory of the focal point and proportional, integral and derivative principles (68). This system claims to be able to make a thermal lesion faster and more stably under real-time thermal monitoring even in moving organs than the existing devices (69).
HIFU therapy has great potential in the field of interventional oncology considering its non-invasiveness and sharp treatment margins leading to treatments with very low complication rates. In addition, uses of this technology in combination with other therapeutic and diagnostic modalities, such as targeted drug/gene-delivery, robotic surgery, and molecular imaging, can be anticipated and has a more revolutionary clinical impact.
It may be too early to predict the future of HIFU therapy. However, there is no doubt that clinical HIFU therapy, at this point in time, is still in its infancy.
Acknowledgment
We thank Kullervo Hynynen, PhD at the department of medical biophysics, University of Toronto for his comments on this work.
References
1. Wood RW, Loomis AL. The physical and biological effects of high frequency sound waves of great intensity. Philos Mag. 1927; 4:417.
2. Feril LB Jr, Kondo T. Biological effects of low intensity ultrasound: the mechanism involved, and its implications on therapy and on biosafety of ultrasound. J Radiat Res. 2004; 45:479–489. PMID: 15635256.
3. Lynn JG, Zwemer RL, Chick AJ, Miller AF. A new method for the generation and use of focused ultrasound in experimental biology. J Gen Physiol. 1942; 26:179–193. PMID: 19873337.


4. Fry WJ, Barnard JW, Fry FJ, Brennan JF. Ultrasonically produced localized selective lesions in the central nervous system. Am J Phys Med. 1955; 34:413–423. PMID: 14376518.
5. Fry FJ. Precision high intensity focusing ultrasonic machines for surgery. Am J Phys Med. 1958; 37:152–156. PMID: 13545382.


6. Fry WJ, Fry FJ. Fundamental neurological research and human neurosurgery using intense ultrasound. IRE Trans Med Electron. 1960; ME-7:166–181. PMID: 13702332.


7. Madersbacher S, Pedevilla M, Vingers L, Susani M, Marberger M. Effect of high-intensity focused ultrasound on human prostate cancer in vivo. Cancer Res. 1995; 55:3346–3351. PMID: 7542168.
8. Dalecki D. Mechanical bioeffects of ultrasound. Annu Rev Biomed Eng. 2004; 6:229–248. PMID: 15255769.


9. American institute of ultrasound in medicine, bioeffects committee. Bioeffects consideration of the safety of diagnostic ultrasound. J Ultrasound Med. 1988; 7:S1–S38. PMID: 3054148.
10. Crocker MJ. Encyclopedia of acoustics. 1997. 1st ed. New York: John Wiley & Sons;p. 6.
11. Nyborg WL. Wu J, Nyborg WL, editors. Mechanisms for bioeffects of ultrasound relevant to therapeutic applications. Emerging therapeutic ultrasound. 2006. 1st ed. Singapore: World Scientific Publishing;p. 5–67.


12. ter Haar GR. Therapeutic application of ultrasound. Prog Biophys Mol Biol. 2007; 93:111–129. PMID: 16930682.
13. ter Haar GR. Hill CR, Bamber JC, ter Haar GR, editors. Therapeutic and surgical applications. Physical principles of medical ultrasound. 2004. 2nd ed. West Sussex: John Wiley & Sons;p. 407–456.


14. Vaezy S, Andrew M, Kaczkowski P, Crum L. Image-guided acoustic therapy. Annu Rev Biomed Eng. 2001; 3:375–390. PMID: 11447068.


15. Hynynen K, McDannold N. Wu J, Nyborg WL, editors. MRI-guided focused ultrasound for local tissue ablation and other image-guided interventions. Emerging therapeutic ultrasound. 2006. 1st ed. Singapore: World Scientific Publishing;p. 167–218.


16. ter Haar GR. Hill CR, Bamber JC, ter Haar GR, editors. Ultrasonic biophysics. Physical principles of medical ultrasound. 2004. 2nd ed. West Sussex: John Wiley & Sons;p. 348–406.


17. Kennedy JE, ter Haar GR, Cranston D. High intensity focused ultrasound: surgery of the future? Br J Radiol. 2003; 76:590–599. PMID: 14500272.


18. Crocker MJ. Encyclopedia of acoustics. 1997. 1st ed. New York: John Wiley & Sons;p. 1727.
19. Biological Effects of Ultrasound: Mechanisms and Clinical Implications, NCRP Report #74. 1983. National Council on Radiation Protection and Measurements (NCRP).
20. Leslie TA, Kennedy JE. High-intensity focused ultrasound principles, current uses, and potential for the future. Ultrasound Q. 2006; 22:263–272. PMID: 17146334.


21. Clement GT. Perspectives in clinical uses of high-intensity focused ultrasound. Ultrasonics. 2004; 42:1087–1093. PMID: 15234170.


22. ter Haar GR. High intensity focused ultrasound for the treatment of tumors. Echocardiography. 2001; 18:317–322. PMID: 11415504.


23. ter Haar GR, Robertson D. Tissue destruction with focused ultrasound in vivo. Eur Urol. 1993; 23:S8–S11.
24. Bush NL, Rivens I, ter Haar GR, Bamber JC. Acoustic properties of lesions generated with an ultrasound therapy system. Ultrasound Med Biol. 1993; 19:789–801. PMID: 8134979.


25. Vaezy S, Martin R, Crum L. High intensity focused ultrasound: a method of hemostasis. Echocardiography. 2001; 18:309–315. PMID: 11415503.
26. Poliachik SL, Chandler WL, Mourad PD, Bailey MR, Bloch S, Cleveland RO, et al. Effect of high-intensity focused ultrasound on whole blood with and without microbubble contrast agent. Ultrasound Med Biol. 1999; 25:991–998. PMID: 10461729.


27. Tachibana S, Koga K. Ultrasound boosting effect to thrombolysis. Blood Vessel. 1981; 12:450–453.
28. Tachibana S. Vibration for boosting fibrinolytic effect of urokinase. Thromb Haemost. 1981; 46(Suppl.):211A.
29. Tachibana K, Tachibana S. The use of ultrasound for drug delivery. Echocardiography. 2001; 18:323–328. PMID: 11415505.


30. Alexandrov AV, Molina CA, Grotta JC, Garami Z, Ford SR, Alvarez-Sabin J, et al. Ultrasound-enhanced systemic thrombolysis for acute ischemic stroke. New Eng J Med. 2004; 351:2170–2178. PMID: 15548777.


31. Liu Y, Yang H, Sakanishi A. Ultrasound: mechanical gene transfer into plant cells by sonoporation. Biotechnol Adv. 2006; 24:1–16. PMID: 15935607.


32. Miller DL, Pislaru SV, Greenleaf JE. Sonoporation: mechanical DNA delivery by ultrasonic cavitation. Somat Cell Mol Genet. 2002; 27:115–134. PMID: 12774945.
33. Jolez FA, Hynynen K. Magnetic resonance image-guided focused ultrasound surgery. Cancer J. 2002; 8:S100–S112. PMID: 12075696.
34. Hynynen K, McDannold N, Clement G, Jolesz FA, Zadicario E, Killiany R, et al. Pre-clinical testing of a phased array ultrasound system for MRI-guided noninvasive surgery of the brain? A primate study. Eur J Radiol. 2006; 59:149–156. PMID: 16716552.
35. Hynynen K, McDannold N, Vykhodtseva N, Jolez FA. Noninvasive MR imaging-guided focal opening of the blood-brain barrier in rabbits. Radiology. 2001; 220:640–646. PMID: 11526261.


36. Yu T, Wang G, Hu K, Ma P, Bai J, Wang Z. A microbubble agent improves the therapeutic efficiency of high intensity focused ultrasound: a rabbit kidney study. Urol Res. 2004; 32:14–19. PMID: 14655029.


37. Kaneko Y, Maruyama T, Takegami K, Watanabe T, Mitsui H, Hanajiri K, et al. Use of a microbubble agent to increase the effects of high intensity focused ultrasound on liver tissue. Eur Radiol. 2005; 15:1415–1420. PMID: 15739112.


38. Hanajiri K, Maruyama T, Kaneko Y, Mitsui H, Watanabe S, Sata M, et al. Microbubble-induced increase in ablation of liver tumors by high-intensity focused ultrasound. Hepatol Res. 2006; 36:308–314. PMID: 16990046.


39. Tachibana K, Tachibana S. Albumin microbubble echo-contrast material as an enhancer for ultrasound accelerated thrombolysis. Circulation. 1995; 92:1148–1150. PMID: 7648659.


40. Molina CA, Ribo M, Rubiera M, Montaner J, Santamarina E, Delgado-Mederos R, et al. Microbubble administration accelerates clot lysis during continuous 2-MHz ultrasound monitoring in stroke patients treated with intravenous tissue plasminogen activator. Stroke. 2006; 37:425–429. PMID: 16373632.


41. Hwang JH, Brayman AA, Reidy MA, Matula TJ, Kimmey MB, Crum LA. Vascular effects induced by combined 1-MHz ultrasound and microbubble contrast agent treatments in vivo. Ultrasound Med Biol. 2005; 31:553–564. PMID: 15831334.


42. Zderic V, Brayman AA, Sharar SR, Crum LA, Vaezy S. Microbubble-enhanced hemorrhage control using high intensity focused ultrasound. Ultrasonics. 2006; 45:113–120. PMID: 16949630.


43. McDannold NJ, Vykhodtseva NI, Hynynen K. Microbubble contrast agent with focused ultrasound to create brain lesions at low power levels: MR imaging and histologic study in rabbits. Radiology. 2006; 241:95–106. PMID: 16990673.


44. Hynynen K, McDannold N, Vykhodtseva N, Jolesz FA. Non-invasive opening of BBB by focused ultrasound. Acta Neurochi Suppl. 2003; 86:555–558.


45. Rabkin BA, Zderic V, Vaezy S. Hyperecho in ultrasound images of HIFU therapy: involvement of cavitation. Ultrasound Med Biol. 2005; 31:947–956. PMID: 15972200.


46. Rabkin BA, Zderic V, Crum LA, Vaezy S. Biological and physical mechanisms of HIFU-induced hyperecho in ultrasound images. Ultrasound Med Biol. 2006; 32:1721–1729. PMID: 17112958.


47. Qian ZW, Xiong L, Yu J, Shao D, Zhu H, Wu X. Noninvasive thermometer for HIFU and its scaling. Ultrasonics. 2006; 44S:E31–E35. PMID: 16844165.


48. Souchon R, Rouviere O, Gelet A, Detti V, Srinivasan S, Ophir J, et al. Visualisation of HIFU lesion using elastography of the human prostate in vivo: preliminary results. Ultrasound Med Biol. 2003; 29:1007–1015. PMID: 12878247.
49. Hindman JC. Proton resonance shift of water in the gas and liquid states. J Chem Phys. 1996; 44:4582–4592.


50. Blana A, Walter B, Rogenhofer S, Wieland WF. High-intensity focused ultrasound for the treatment of localized prostate cancer: 5-year experience. Urology. 2004; 63:297–300. PMID: 14972475.


51. Uchida T, Ohkusa H, Yamashita H, Shoji S, Nagata Y, Hyodo T, et al. Five years experience of transrectal high-intensity focused ultrasound using the Sonablate device in the treatment of localized prostate cancer. Int J Urol. 2006; 13:228–233. PMID: 16643614.


52. Poissonnier L, Chapelon JY, Rouviere O, Curiel L, Bouvier R, Martin X, et al. Control of prostate cancer by transrectal HIFU in 227 patients. Eur Urol. 2007; 51:381–387. PMID: 16857310.


53. Gelet A, Chapelon JY, Poissonnier L, Bouvier R, Rouviere O, Curiel L, et al. Local recurrence of prostate cancer after external beam radiotherapy: early experience of salvage therapy using high-intensity focused ultrasonography. Urology. 2004; 63:625–629. PMID: 15072864.


54. Stewart EA, Rabinovici J, Tempany CM, Inbar Y, Regan L, Gostout B, et al. Clinical outcomes of focused ultrasound surgery for the treatment of uterine fibroids. Fertil Steril. 2006; 85:22–29. PMID: 16412721.


55. Hindley J, Gedroyc WM, Regan L, Stewart E, Tempany C, Hynynen K, et al. MRI guidance of focused ultrasound therapy of uterine fibroid: early results. AJR Am J Roentgenol. 2004; 183:1713–1719. PMID: 15547216.
56. Smart OC, Hindley JT, Regan L, Gedroyc WG. Gonadotrophin-releasing hormone and magnetic-resonance-guided ultrasound surgery for uterine leiomyomata. Obstet Gynecol. 2006; 108:49–54. PMID: 16816055.


57. Wu F, Wang ZB, Zhu H, Chen WZ, Zou JZ, Bai J, et al. Extracorporeal high intensity focused ultrasound treatment for patients with breast cancer. Breast Cancer Res Treat. 2005; 92:51–60. PMID: 15980991.


58. Furusawa H, Namba K, Thomsen S, Akiyama F, Bendet A, Tanaka C, et al. Magnetic resonance-guided focused ultrasound surgery of breast cancer: reliability and effectiveness. J Am Coll Surg. 2006; 203:54–63. PMID: 16798487.


59. Kennedy JE, Wu F, ter Haar GR, Gleeson FV, Phillips RR, Middleton MR, et al. High-intensity focused ultrasound for the treatment of liver tumors. Ultrasonics. 2004; 42:931–935. PMID: 15047409.
60. Wu F, Wang ZB, Chen WZ, Zhu H, Bai J, Zou JZ, et al. Extracorporeal high intensity focused ultrasound ablation in the treatment of patients with large hepatocellular carcinoma. Ann Surg Oncol. 2004; 11:1061–1069. PMID: 15545506.


61. Wu F, Wang ZB, Chen WZ, Zou JZ, Bai J, Zhu H, et al. Advanced hepatocellular carcinoma: treatment with high-intensity focused ultrasound ablation combined with transcatheter arterial embolization. Radiology. 2005; 235:659–667. PMID: 15858105.


62. Wu F, Wang ZB, Zhu H, Chen WZ, Zou JZ, Bai J, et al. Feasibility of US-guided high-intensity focused ultrasound treatment in patients with advanced pancreatic cancer: initial experience. Radiology. 2005; 236:1034–1040. PMID: 16055692.


63. Wu F, Wang ZB, Chen WZ, Bai J, Zhu H, Qiao TY. Preliminary experience using high intensity focused ultrasound for the treatment of patients with advanced stage renal malignancy. J Urol. 2003; 170:2237–2240. PMID: 14634387.


64. Wu F, Wang ZB, Chen WZ, Wang W, Gui Y, Zhang M, et al. Extracorporeal high intensity focused ultrasound ablation in the treatment of 1038 patients with solid carcinomas in China: an overview. Ultrason Sonochem. 2004; 11:149–154. PMID: 15081972.


65. Kratzik C, Schatzl G, Lackner J, Marberger M. Transcutaneous high-intensity focused ultrasonography can cure testicular cancer in solitary testis. Urololgy. 2006; 67:1269–1273.


66. Ram Z, Cohen ZR, Harnof S, Tal S, Faibel M, Nass D, et al. Magnetic resonance imaging-guided, high-intensity focused ultrasound for brain tumor therapy. Neurosurgery. 2006; 59:949–956. PMID: 17143231.
67. Li JJ, Xu GL, Gu MF, Luo GY, Rong Z, Wu PH, et al. Complications of high intensity focused ultrasound in patients with recurrent and metastatic abdominal tumors. World J Gastroenterol. 2007; 13:2747–2751. PMID: 17569147.


68. Mougenot C, Salomir R, Palussiere J, Grenier N, Moonen CT. Automatic spatial and temporal temperature control for MR-guided focused ultrasound using fast 3D MR thermometry and multispiral trajectory of the focal point. Magn Reson Med. 2004; 52:1005–1015. PMID: 15508173.


69. de Senneville BD, Mougenot C, Moonen CT. Real-time adaptive method for treatment of mobile organs by MRI-controlled high-intensity focused ultrasound. Magn Reson Med. 2007; 57:319–330. PMID: 17260361.
Fig. 2
Basic concept of HIFU-induced tissue change by hyperthermia. As US waves are focused onto small spot, acoustic pressure is rapidly elevated near focus where tissue temperatures are also raised to level that is sufficient for thermotherapeutic effects, resulting in coagulation necrosis.
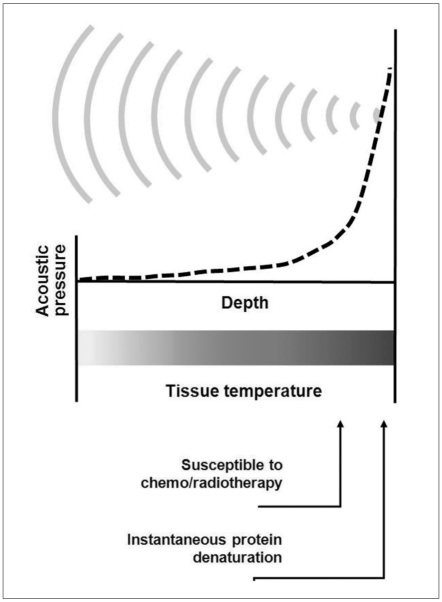
Fig. 3
Relationships between sound pressure, power, energy, and intensity. Sonic intensity, defined as energy passing through unit area within unit time, is derived from plane wave. As seen in equation, intensity is proportional to square of acoustic pressure and is also function of property of medium (density and speed of sound) through which waves are propagated.
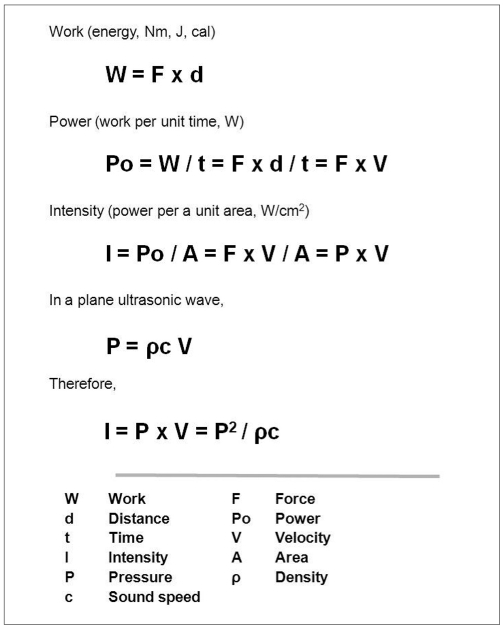
Fig. 4
Various methods of focusing US waves: A. Spherically-curved transducer, B. Flat transducer with interchangeable lens, C. Phased-array transducer causing only steering, and D. Phased-array transducer causing steering and focusing at same time.
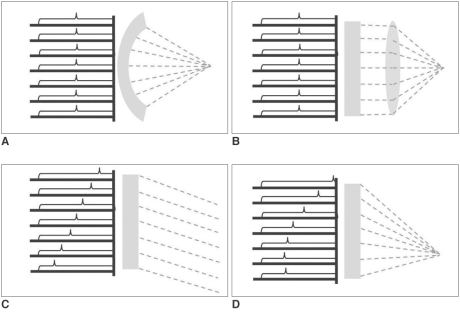
Fig. 5
Classical thermal lesion formed by focused US surgery (US absorption only) on porcine liver specimen.
A. Cigar-shaped thermal lesion is formed at focal zone of US wave pathway (two overlaid triangles) following HIFU single exposure.
B. Final thermal lesion after stacking each single lesion. Single lesions are much smaller than clinically common tumors and therefore each thermal lesion should be stacked compactly without leaving intervening viable tissue. This lesion can cover entire pathological lesion as well as has very sharp margin that could be controlled easily.
