Abstract
Atherosclerosis is an inflammatory disease as well as a lipid disorder. Atherosclerotic plaque formed in vessel walls may cause ischemia, and the rupture of vulnerable plaque may result in fatal events, like myocardial infarction or stroke. Because morphological imaging has limitations in diagnosing vulnerable plaque, molecular imaging has been developed, in particular, the use of nuclear imaging probes. Molecular imaging targets various aspects of vulnerable plaque, such as inflammatory cell accumulation, endothelial activation, proteolysis, neoangiogenesis, hypoxia, apoptosis, and calcification. Many preclinical and clinical studies have been conducted with various imaging probes and some of them have exhibited promising results. Despite some limitations in imaging technology, molecular imaging is expected to be used both in the research and clinical fields as imaging instruments become more advanced.
Atherosclerosis is a disease condition where lipid-rich atheroma is formed in the vessel wall. It results in arterial luminal narrowing, and symptomatic atherosclerosis may cause impaired blood flow to the subtended organs, resulting in angina or a transient ischemic attack of the brain. Additionally, more critical adverse events, such as myocardial infarction or stroke can occur from a thromboembolism resulting from atheroma. Cardiovascular disease and stroke were the causes of death in 9.5% and 9.6% of all deaths, in Korea in 2013 (1), respectively, and the rates were 14.9% and 5.1%, respectively, in the United States in 2011 (2). However, fatal events from atherosclerosis occur only in a certain portion of atherosclerosis. Although atherosclerosis is observed in 85% of the elderly over the age of 50 years, and in 1 of 6 in adolescents (3), it does not always cause fatal complications. On the other hand, in many cases of myocardial infarction, culprit lesions of coronary artery do not show significant arterial narrowing (4). Fatal myocardial infarctions or sudden cardiac death have been presented as the first manifestation of coronary artery disease without preceding ischemic symptoms in 62% of male and 42% of female patients (5). Thus, vulnerable atherosclerotic plaque, also called 'active-forming' or 'rupture-prone' plaque, needs to be discriminated from stable plaques.
Atherosclerosis has been one of the most actively investigated fields in medical imaging. Atherosclerosis can be evaluated by using various imaging methods. Tissue perfusion, which can be evaluated on single photon emission computed tomography (SPECT), computed tomography (CT), and magnetic resonance imaging (MRI), has been deemed as a functional imaging tool for atherosclerosis. More direct imaging of atherosclerosis is available by using angiography, CT, and MRI. Additionally, ultrasonography also may be used to evaluate atherosclerosis in some specific arteries, including the carotid artery. However, these imaging methods are based on the anatomical aspects of atherosclerosis and can hardly identify the activity and vulnerability of an atherosclerotic lesion, although information on plaque composition can be partially identified on CT or MRI. Recently, there have been many advancements in molecular imaging, which visualizes specific molecular biomarkers or molecular processes in vivo. Various molecular imaging methods have been developed in cancer imaging to identify molecular characteristics of tumors. These methods can be applied to vulnerable plaque, which has similar imaging targets.
Molecular imaging usually utilizes specific imaging probes, and thus, nuclear imaging with gamma camera or positron emission tomography (PET) has an advantage over CT, MRI, and ultrasonography, in that it has flexibility in designing various imaging probes. There have been many studies in which nuclear molecular imaging was applied to vulnerable plaques. In this article, various molecular mechanisms and nuclear imaging methods for vulnerable plaque are reviewed and considerations regarding the technical aspects of those imaging methods are briefly discussed.
Atherosclerosis is an inflammatory disease as well as a lipid disorder. Lipid, the core component of a plaque, is deposited in the arterial wall under hyperlipidemic conditions. Low-density lipoprotein (LDL) is the most important factor in the initiation of atherosclerosis because it moves through the arterial endothelium by diffusion. LDL may exhibit spontaneous involution; however, LDL is prone to oxidation when it is isolated from circulating antioxidants (67). The oxidation process converts LDL into minimally modified LDL, oxidized LDL, and glycated LDL. These converted LDLs, particularly oxidized LDL, stimulate endothelial cells (EC) and macrophages through CD14 and toll-like receptor, eventually evoking inflammatory processes (89). Accordingly, vascular ECs express several adhesion molecules, such as selectin (CD62) and vascular cell adhesion molecule (VCAM)-1 (10), which are necessary for circulating leukocytes to roll on or to attach to the endothelium (Fig. 1). Monocytes and macrophages release proteases including matrix metalloproteinase (MMP) and cathepsin for easier cell migration in the inflammatory tissue (1112). In migrated macrophages, scavenger receptor expression is enhanced so that macrophages can engulf LDL derivatives through receptor-mediated endocytosis, and the lipid-laden macrophages are called 'foam cells'. Macrophages also release proinflammatory cytokines such as tumor necrosis factor-α and interleukin (IL)-1β to amplify inflammation. Foam cells are characteristic cells in the initial process of atherosclerosis.
With the progression of atherosclerosis, plaques are loosened by several proteases that are released by macrophages, so that vascular smooth muscle cells (SMC) migrate into the plaque. SMCs produce collagens to fill the extracellular matrix, and the fibrous tissue deposition is a characteristic feature of atherosclerosis progression. In this process, IL-18, interferon (IFN)-γ, and CD40L work as critical cytokines affecting most of the inflammation-involved cells including ECs, macrophages, T-cells, SMCs, and platelets (13). In vulnerable atherosclerotic plaques, active and prolonged inflammation leads to hypoxia in the local tissue and neoangiogenesis in the plaque (14). Newly developed vessels in the plaque are pathways for leukocytes to migrate into the plaque to stimulate further inflammation. However, these new vessels are fragile and prone to hemorrhage, which results in abrupt plaque enlargement and the generation of thrombin (15). Apoptosis of inflammation-involved cells occurs in the vulnerable plaques and microcalcification follows as a type of healing process.
Plaque rupture is the key event for fatal complications of atherosclerosis. The rupture is caused by progressed inflammation in the plaque. IFN-γ released by helper T-cells inhibits collagen production by SMCs, while IL-1 and CD40L promote the release of collagenase and MMP from macrophages (13). All these cytokines, as well as intraplaque hemorrhage and necrosis, lead to thinning, erosion, and finally, disruption of the fibrous cap, which causes contact between the highly thrombogenic plaque with blood in the arterial lumen. Afterward, thrombus formation is rapidly promoted and the unstable or ruptured thrombus may cause an embolism in distal part of the artery. A thrombus with a thin stalk can be a direct cause of fatal complications, such as acute coronary syndrome and cerebral infarction.
Most of above-mentioned pathologic processes in vulnerable plaques have been selected as targets in molecular imaging. Among them, recently reported studies are summarized in Table 1.
The first step of atherosclerosis is the accumulation of LDL or its oxidized derivatives, which was adopted as a target for vulnerable plaque imaging in early studies (16171819). However, the imaging methods did not appear to be effective enough for subsequent studies.
Endothelial activation is the beginning of inflammation. The expression of selectins or VCAM-1 on the activated endothelium has been selected as an imaging target, and compounds mimicking sialyl-lewis X (SLX), a natural leukocyte receptor for selectins, or antibodies against selectin or VCAM-1 were utilized for imaging probes. Because an antibody usually exhibits a long half-life in the circulation of a living body, it needs to be labeled by radioisotopes with long half-lives. Recently, a monoclonal antibody for P-selectin labeled with 64Cu (T1/2 = 12.7 hours) was reported to be effective for vulnerable plaque imaging in an animal study (20). A small antibody fragment for VCAM-1 (21) and peptide compounds for VCAM-1 (22) have relatively shorter half-lives in circulation, and have been labeled with 99mTc and 18F, respectively. They also exhibited promising results in plaque imaging. Fucoidan is a synthetic SLX-mimicking compound, and increased 68Ga-fucoidan uptake in atherosclerotic plaques in a proof-of-concept study (23). Monocyte recruitment and migration in atherosclerotic plaques may be imaged by the direct radiolabeling of cells (24).
The most extensively investigated imaging targets in vulnerable plaques are activated macrophages, and fluorodeoxyglucose (FDG) is the most widely used imaging probe in inflammation (Fig. 2). Although almost all inflammatory cells exhibit increased glucose metabolism, macrophages are the most important cells regarding FDG uptake. There is a close correlation between FDG uptake and macrophage accumulation, both in animal models (252627) and human studies (282930). FDG uptake is observed chiefly in the necrotic lipid core, when it is correlated with MRI (31). As macrophages are key players in the inflammatory process, FDG uptake is also correlated with systemic inflammatory markers, such as serum MMP-1 level (32). FDG uptake is also related to other risk factors for fatal atherosclerotic diseases; carotid intima-media thickness, serum high-density lipoprotein, C-reactive protein (3334), smoking, hypertension, hypercholesterolemia, and age (3536).
Arterial calcification detected on CT is evidence of inflammation, and coronary calcification is considered to be a risk factor for coronary events. However, it has been repeatedly reported that arterial calcification does not always accord with FDG uptake (373839), probably because FDG uptake reflects the present activity whereas calcifications on CT are the end-products of inflammation. Consequently, FDG uptake is related to cardiovascular events or stroke (4041), and probably is a better predictor for vascular events than calcifications on CT (42). Based on this background, FDG PET has been used for assessing inflammation activity in the case of drug treatment (4344). Like these studies, therapeutic efficacy monitoring is one promising application for vulnerable plaque imaging.
Fluorodeoxyglucose is an excellent imaging agent for clinical applications because it is already approved in many countries and widely used in clinical practice. However, myocardial FDG uptake varies from patient to patient despite its standard preparation protocol (Fig. 3) (45), and thus, FDG PET is considerably limited with respect to the evaluation of coronary arteries due to its variable background activity. Although some protocols have been suggested to reduce physiological FDG uptake in the myocardium, including extended fasting (46), heparin injection (47), and low-carbohydrate high-fat diet (48), other imaging methods are required for evaluating vulnerable plaques in coronary arteries.
In addition to FDG, many other vulnerable plaque imaging methods are targeting activated macrophages. Surface receptors expressed on macrophages were selected as imaging targets in early studies. Imaging probes for the IL-2 receptor (49) and scavenger receptors (5051) were labeled with 99mTc and tested in plaque imaging more than a decade ago. 99mTc-HYNIC-IL-2 is another IL-2 receptor targeting probe and recently has been utilized in a human study for carotid plaque imaging (52). In more recent studies, chemokine receptors have been investigated. A viral macrophage inflammatory protein II that has an affinity for chemokine receptors was labeled with 64Cu and used for PET imaging in a mouse model (53). A peptide moiety of D-Ala1-peptide T-amide (DAPTA), which has an affinity for chemokine receptors, was also labeled with 64Cu after conjugation with a comb-like nanoparticle. 64Cu-DAPTA-comb was reported to be an effective imaging probe for atherosclerosis (54).
There are other surface receptors of macrophages that have been utilized for plaque imaging. Activated macrophages express folate receptor-β, for which 111In-labeled folate was designed. 111In-labeled folate SPECT/CT successfully showed vulnerable plaques in a mouse model (55). Intriguingly, folate receptor-β was reported to be overexpressed on the M2-subtype of macrophages rather than on the M1-subtype (56). The mannose receptor is also expressed on activated macrophages, particularly the M2-subtype, and its use has been attempted in plaque imaging with 18F-fluoro-D-mannose (57). Other surface receptors of macrophages, such as lectin-like oxidized LDL receptor (LOX)-1 (58) and somatostatin (SST) receptors (596061) have been attempted for plaque imaging by using 111In-labeled antibody against LOX-1 or 68Ga-DOTA-(Tyr3)-octreotate (DOTATATE). Among them, 68Ga-DOTATATE can readily be used for human studies because it has long been used for human imaging for SST receptor-expressing neuroendocrine tumors.
Translocator protein (TSPO), formerly called the peripheral benzodiazepine receptor, is a membrane protein abundantly found on the outer mitochondria membrane as well as the surface of activated macrophage and mast cells. There are several compounds that have affinity for TSPO, such as PBR28 and PK11195. 3H or 11C labeled PK11195 has exhibited a high binding affinity for the atherosclerotic plaque (6263). 11C-PK11195 has already been used in human studies (6465), which have also exhibited the feasibility of 11C-PK11195 PET for vulnerable plaque imaging. However, TSPO has a limitation because normal myocardium expresses TSPO and coronary imaging is difficult due to a high level of background activity (Fig. 4).
Choline is used for cell membrane synthesis and choline metabolism is enhanced in activated macrophages. Thus, radiolabeled choline is taken up highly in atherosclerotic lesions (66). 11C-choline and 18F-choline is available for human application and PET imaging is often performed in prostate cancer patients. Thus, plaque imaging studies using these agents have been conducted in humans and the high uptake by the plaque has been reported (6768). Non-specific 64Cu-nanoparticle also exhibits a high uptake in a plaque lesion, because of its enhanced permeability and retention effect in the inflammation area and phagocytosis by macrophages (69).
Proteolysis is mediated by several proteases that are released by macrophages. It is a requisite process to loosen extracellular space so that inflammatory cells and smooth muscle cells migrate through the space. In inflammatory proteolysis, MMP and cathepsin are most important proteases, and radiolabeled imaging tracers for MMP have been developed. In early studies, MMP inhibitors labeled with 123I or 99mTc were used for scintigraphic scan and SPECT, and showed increased uptake in the injured or atherosclerotic arteries (7071). For PET imaging, an MMP inhibitor labeled with 18F was also developed and tested in a mouse model (72). More recently, 99mTc-RP805 that has a specific affinity for active MMP has been attempted for vulnerable plaque imaging in animal models (7374).
Neoangiogenesis in plaques is another hallmark of vulnerable plaque. Neoangiogenesis-targeted imaging methods comprise two main groups; one group targeting integrin αvβ3 and the other targeting vascular endothelial growth factor (VEGF) receptors. Integrin is a group of cellular transmembrane proteins that has two main functions; intercellular attachment and intracellular signal transduction. Integrin is composed of two subunits α and β, which make many subtypes of integrin. As an example, β2 chain is a main component of integrin subtypes on circulating monocytes and lymphocytes, which binds to VCAM-1 or intercellular adhesion molecule-1 on the surface of vascular endothelium in an inflammation site.
Integrin αvβ3, a receptor for fibronectin and vitronectin, is expressed on activated endothelium and turn on angiogenic program by cross-talking with various growth factor receptors (75). Intriguingly, a tri-peptide moiety of Arg-Gly-Asp (RGD) has a high affinity for integrin αvβ3 and has been utilized for angiogenesis imaging. 99mTc-NC100692, one of radiolabeled RGD compounds, exhibited high uptake in the atherosclerotic carotid artery in an animal model (76). For PET, 68Ga-NOTA-RGD has been tested in vulnerable plaque imaging (77). 18F is the most widely used radioisotope for PET imaging and 18F-flotegatide and 18F-galactoRGD have also been tested for plaque imaging (787980). Because RGD is a simple and safe functional moiety, 68Ga-NOTA-RGD and 18F-galactoRGD can be readily applied to human studies (777981). However, it should be noted that integrin αvβ3 is expressed on macrophages as well as activated endothelium, and thus, this imaging visualizes both neoangiogenesis and macrophage accumulation in vulnerable plaques. VEGF receptor that is expressed on activated endothelium is another target for neoangiogenesis imaging. Recently, an antibody against VEGF receptor was labeled with 89Zr and tested in carotid plaque PET imaging (82).
Hypoxia in the plaque results from increase in plaque size and inflammation-related metabolic activation in plaques. Thus, hypoxia is another hallmark in vulnerable plaques. 18F-fluoromisonidazole (FMISO) is a derivative of nitroimidazole and widely used for hypoxia imaging. In hypoxic conditions, nitroimidazole is reduced and R-NO2 is converted into R-NH2, resulting in cellular retention (83). Most 18F-FMISO imaging has been used to investigate tumors; however, it was also reported that vulnerable plaques can be visualized using 18F-FMISO PET in an animal model (84). Apoptosis in the plaque occurs in macrophages or other cells as a result of active inflammation. Apoptosis can be visualized by radiolabeled annexin V, which binds to phosphatidylserine on the apoptotic cells' surfaces. In early studies, 99mTc-annexin V exhibited high uptake in atherosclerotic plaques (858687).
Calcification is a final process in inflammation. Although calcified plaques can be easily visualized on CT, current calcifying activity in the plaque is not reflected on CT. The calcifying activity can be imaged by using a 99mTc-phosphonate scan or 18F-fluoride PET. Notably, these imaging methods have long been used in clinical practice to assess bone metabolism or bone lesions. Thus, nearly all 18F-fluoride PET studies in vascular disorders have been conducted in humans (888990919293), not in animal models. 18F-fluoride uptake in a plaque is not always related to calcification on CT (Fig. 5). Additionally, 18F-fluoride PET exhibits significantly higher uptake in symptomatic or ruptured plaques than in stable plaques, and is related to other pathologic markers for vulnerable plaques, such as necrotic cores (89).
Quantifying ability is one of the important advantages of nuclear imaging, and the standardized uptake value (SUV) is most widely utilized as a quantitative index in PET imaging for cancers. In FDG PET for plaque imaging, SUV measurements exhibited high reproducibility when FDG PET was repeated with certain time intervals (949596). However, there is no consensus for the selection of an appropriate quantitative index for atherosclerosis, in contrast to cancers where the maximal SUV is commonly utilized as an index reflecting the most malignant components of a lesion. Among reported FDG PET studies on plaque imaging, some adopted the maximal SUV (3238394142), whereas others adopted the mean SUV as a quantitative index (33364094). Based on these values, additional semi-quantitative indices were calculated, such as the target-to-reference tissue ratio (TRR; also called target-to-background ratio, TBR) or the lesion-to-normal ratio (LNR) (Table 1). Usually, a vein, muscle, and the lungs are selected as reference organs, and a non-atherosclerotic or asymptomatic artery is selected as normal tissue. In plaque imaging, the maximal SUV (or TRR, TBR, or LNR) may reflect the most active and vulnerable component of a lesion, whereas the mean SUV of all plaque lesions may reflect the overall atherosclerotic activity of a patient. The quantitative index needs to be optimized based on the purpose of each study, and further studies are needed to determine the most effective quantitative index.
In spite of some promising results, there are still considerable limitations in nuclear molecular imaging methods for the evaluation of vulnerable plaques. First of all, the accumulation of an imaging probe and signal are not enough in a target lesion because most target arteries are small and easily affected by the partial volume effect. Thus, the LNR is less than 2.0 in human imaging studies, whereas it is up to 6.0-7.0 in small animal or human tissue studies (Table 1). Motion artifacts from respiration or pulsation may also affect image quality. In particular, coronary arteries are more prone to motion artifacts caused by cardiac contractions, while they are one of the most important targets in vulnerable plaque imaging. Respiratory and electrocardiography-gated image acquisition can be a solution for these motion artifacts. However, gated acquisition results in a low signal, and consequently, poor image quality. Highly sensitive nuclear imaging instruments are currently under active development, and image quality is expected to improve in the near future, even in small lesions, such as atherosclerotic plaques.
Vulnerable plaque is the direct cause of fatal atherosclerotic diseases. While current morphological imaging methods have limitations in diagnosing vulnerable plaque, molecular imaging methods can be alternative diagnostic tools. Currently, there are many molecular imaging probes for SPECT or PET, targeting diverse aspects of vulnerable plaque, such as inflammatory cells, endothelial activation, proteolysis, neoangiogenesis, hypoxia, apoptosis, and calcification. Although these imaging methods have several technical limitations at present, it is expected that they can be used both in research and clinical fields as advancements in imaging technology are made.
Figures and Tables
Fig. 2
Arterial fluorodeoxyglucose (FDG) uptake related to atherosclerosis.
On positron emission tomography (PET) coronal (A), PET/CT fusion coronal (B), and transaxial (C) images of 68-year-old man, focal increased FDG uptake is observed in right brachiocephalic artery (arrows). Increased FDG uptake is often incidentally observed in major arteries during diagnostic workup for cancer. FDG uptake is sometimes very strong, whereas FDG uptake is also observed in other arteries to certain degree (arrowhead).
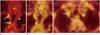
Fig. 3
Variable physiological fluorodeoxyglucose (FDG) uptake in heart.
FDG uptake is not suppressed despite standard 6-hour fasting (A), whereas uptake is completely suppressed by same preparation protocol (B).
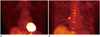
Fig. 4
Translocator protein (TSPO)-targeted imaging in rat model.
On transaxial (A) and coronal (B) images of 11C-PBR28 fusion positron emission tomography/CT, increased uptake is observed in whole myocardium. Although TSPO is promising imaging target for inflammation and vulnerable plaque, its role in coronary artery is thought to be limited because of its normal uptake in myocardium.
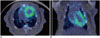
Fig. 5
18F-fluoride positron emission tomography (PET) in atherosclerosis.
In patient with unstable angina, increased 18F-fluoride uptake is observed in left main and left anterior descending artery on PET (A), PET/CT fusion (B), and CT (C) images (arrow). Uptake is well-matched with dense calcification on CT. However, 18F-fluoride uptake is also increased in aortic wall (arrowhead), despite lack of definite calcification on CT.
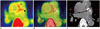
Table 1
Recently Reported Molecular Imaging Methods for Vulnerable Plaques
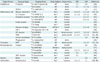
Target Process | Imaging Target | Imaging Probe | Study Subjects | Target Artery | TRR | LNR | References |
---|---|---|---|---|---|---|---|
Endothelium | P-selectin | 64Cu-anti P-selectin Ab | AM | Aorta | 1.3 | 5.9 | (20) |
68Ga-fucoidan | AM | Aorta | 5.1 | 1.7-2.4 | (23) | ||
VCAM-1 | 99mTc-cAbVCAM1-5 | AM | Aorta | 1.3 | (21) | ||
Inflammatory cell | Glucose metabolism | 18F-FDG | Hu-I | Carotid | 1.5-2.2 | 1.1-1.2 | (40, 41) |
IL-2 receptor | 99mTc-HYNIC-IL-2 | Hu-T | Carotid | 1.1-1.6 | 1.2-1.4 | (52) | |
Chemokine receptor | 64Cu-DOTA-vMIP-II | AM | Femoral | 3.4-3.7 | (53) | ||
64Cu-DOTA-DAPTA | AM | Femoral | 2.9-4.4 | (54) | |||
Folate receptor | 111In-EC0800 | AM | Carotid | (55) | |||
99mTc-folate | Hu-T | Carotid | (56) | ||||
Mannose receptor | 18F-fluoro-D-mannose | AM/Hu-T | Carotid | 2.3-4.8 | (57) | ||
LOX-1 | 111In-liposome-LOX-1 Ab | AM | Aorta | (58) | |||
SST receptor | 68Ga-DOTATATE | Hu-I | Aorta/coronary | 1.4-3.7 | 1.2-1.5 | (59, 60, 61) | |
TSPO | 11C-PK11195 | Hu-I | Aorta/carotid | 1.1-2.4 | 1.2-2.5 | (64, 65) | |
Choline metabolism | 18F/11C-choline | Hu-I | 1.9 | (67, 68) | |||
Phagocytosis | 64Cu-nanoparticle | AM | Aorta | (69) | |||
Proteolysis | MMP | 99mTc-RP805 | AM | Aorta/carotid | 1.3-1.7 | (73, 74) | |
Neoangiogenesis | Integrin | 99mTc-NC100692 | AM | Carotid | 6.5 | (76) | |
68Ga-NOTA-RGD | AM/Hu-I | Aorta/carotid | (77) | ||||
18F-flotegatide | AM | Aorta | 1.5-4.7 | 2.8-5.2 | (78) | ||
18F-galactoRGD | Hu-I | Carotid | 2.0 | 1.7 | (79) | ||
VEGF receptor | 89Zr-bevacizumab | Hu-T | Carotid | 2.1 | (82) | ||
Hypoxia | Redox | 18F-FMISO | AM | Aorta | 2.5 | (84) | |
Calcification | Chemisorption | 18F-fluoride | Hu-I | Coronary | 1.3-1.7 | 1.1 | (88, 89) |
Ab = antibody, AM = animal model, Hu-I = human imaging, Hu-T = human tissue specimen, IL = interleukin, LDL = low-density lipoprotein, LNR = lesion-to-normal ratio, LOX = lectin-like oxidized LDL receptor, MMP = matrix metalloproteinase, SST = somatostatin, TRR = target-to-reference tissue ratio, TSPO = translocator protein, VCAM = vascular cell adhesion molecule, VEGF = vascular endothelial growth factor
References
1. KOSTAT. Causes of death statistics in Korean. 2014. 09. 23. Accessed April 23, 2015. http://kostat.go.kr/portal/korea/kor_nw/2/1/index.board?bmode=read&aSeq=330181.
2. Mozaffarian D, Benjamin EJ, Go AS, Arnett DK, Blaha MJ, Cushman M, et al. Heart disease and stroke statistics--2015 update: a report from the American Heart Association. Circulation. 2015; 131:e29–e322.
3. Tuzcu EM, Kapadia SR, Tutar E, Ziada KM, Hobbs RE, McCarthy PM, et al. High prevalence of coronary atherosclerosis in asymptomatic teenagers and young adults: evidence from intravascular ultrasound. Circulation. 2001; 103:2705–2710.
4. Hackett D, Davies G, Maseri A. Pre-existing coronary stenoses in patients with first myocardial infarction are not necessarily severe. Eur Heart J. 1988; 9:1317–1323.
5. Murabito JM, Evans JC, Larson MG, Levy D. Prognosis after the onset of coronary heart disease. An investigation of differences in outcome between the sexes according to initial coronary disease presentation. Circulation. 1993; 88:2548–2555.
6. Skålén K, Gustafsson M, Rydberg EK, Hultén LM, Wiklund O, Innerarity TL, et al. Subendothelial retention of atherogenic lipoproteins in early atherosclerosis. Nature. 2002; 417:750–754.
7. Kruth HS. Sequestration of aggregated low-density lipoproteins by macrophages. Curr Opin Lipidol. 2002; 13:483–488.
8. Glass CK, Witztum JL. Atherosclerosis. the road ahead. Cell. 2001; 104:503–516.
9. Miller YI, Chang MK, Binder CJ, Shaw PX, Witztum JL. Oxidized low density lipoprotein and innate immune receptors. Curr Opin Lipidol. 2003; 14:437–445.
10. Cybulsky MI, Iiyama K, Li H, Zhu S, Chen M, Iiyama M, et al. A major role for VCAM-1, but not ICAM-1, in early atherosclerosis. J Clin Invest. 2001; 107:1255–1262.
11. Amorino GP, Hoover RL. Interactions of monocytic cells with human endothelial cells stimulate monocytic metalloproteinase production. Am J Pathol. 1998; 152:199–207.
12. Mason DP, Kenagy RD, Hasenstab D, Bowen-Pope DF, Seifert RA, Coats S, et al. Matrix metalloproteinase-9 overexpression enhances vascular smooth muscle cell migration and alters remodeling in the injured rat carotid artery. Circ Res. 1999; 85:1179–1185.
13. Sukhova GK, Schönbeck U, Rabkin E, Schoen FJ, Poole AR, Billinghurst RC, et al. Evidence for increased collagenolysis by interstitial collagenases-1 and -3 in vulnerable human atheromatous plaques. Circulation. 1999; 99:2503–2509.
14. Moulton KS, Heller E, Konerding MA, Flynn E, Palinski W, Folkman J. Angiogenesis inhibitors endostatin or TNP-470 reduce intimal neovascularization and plaque growth in apolipoprotein E-deficient mice. Circulation. 1999; 99:1726–1732.
15. Virmani R, Kolodgie FD, Burke AP, Finn AV, Gold HK, Tulenko TN, et al. Atherosclerotic plaque progression and vulnerability to rupture: angiogenesis as a source of intraplaque hemorrhage. Arterioscler Thromb Vasc Biol. 2005; 25:2054–2061.
16. Iuliano L, Signore A, Vallabajosula S, Colavita AR, Camastra C, Ronga G, et al. Preparation and biodistribution of 99m technetium labelled oxidized LDL in man. Atherosclerosis. 1996; 126:131–141.
17. Tsimikas S, Palinski W, Halpern SE, Yeung DW, Curtiss LK, Witztum JL. Radiolabeled MDA2, an oxidation-specific, monoclonal antibody, identifies native atherosclerotic lesions in vivo. J Nucl Cardiol. 1999; 6(1 Pt 1):41–53.
18. Shaw PX, Hörkkö S, Tsimikas S, Chang MK, Palinski W, Silverman GJ, et al. Human-derived anti-oxidized LDL autoantibody blocks uptake of oxidized LDL by macrophages and localizes to atherosclerotic lesions in vivo. Arterioscler Thromb Vasc Biol. 2001; 21:1333–1339.
19. Tekabe Y, Li Q, Rosario R, Sedlar M, Majewski S, Hudson BI, et al. Development of receptor for advanced glycation end products-directed imaging of atherosclerotic plaque in a murine model of spontaneous atherosclerosis. Circ Cardiovasc Imaging. 2008; 1:212–219.
20. Nakamura I, Hasegawa K, Wada Y, Hirase T, Node K, Watanabe Y. Detection of early stage atherosclerotic plaques using PET and CT fusion imaging targeting P-selectin in low density lipoprotein receptor-deficient mice. Biochem Biophys Res Commun. 2013; 433:47–51.
21. Broisat A, Toczek J, Dumas LS, Ahmadi M, Bacot S, Perret P, et al. 99mTc-cAbVCAM1-5 imaging is a sensitive and reproducible tool for the detection of inflamed atherosclerotic lesions in mice. J Nucl Med. 2014; 55:1678–1684.
22. Nahrendorf M, Keliher E, Panizzi P, Zhang H, Hembrador S, Figueiredo JL, et al. 18F-4V for PET-CT imaging of VCAM-1 expression in atherosclerosis. JACC Cardiovasc Imaging. 2009; 2:1213–1222.
23. Li X, Bauer W, Israel I, Kreissl MC, Weirather J, Richter D, et al. Targeting P-selectin by gallium-68-labeled fucoidan positron emission tomography for noninvasive characterization of vulnerable plaques: correlation with in vivo 17.6T MRI. Arterioscler Thromb Vasc Biol. 2014; 34:1661–1667.
24. Kircher MF, Grimm J, Swirski FK, Libby P, Gerszten RE, Allport JR, et al. Noninvasive in vivo imaging of monocyte trafficking to atherosclerotic lesions. Circulation. 2008; 117:388–395.
25. Ogawa M, Ishino S, Mukai T, Asano D, Teramoto N, Watabe H, et al. (18)F-FDG accumulation in atherosclerotic plaques: immunohistochemical and PET imaging study. J Nucl Med. 2004; 45:1245–1250.
26. Aziz K, Berger K, Claycombe K, Huang R, Patel R, Abela GS. Noninvasive detection and localization of vulnerable plaque and arterial thrombosis with computed tomography angiography/positron emission tomography. Circulation. 2008; 117:2061–2070.
27. Davies JR, Izquierdo-Garcia D, Rudd JH, Figg N, Richards HK, Bird JL, et al. FDG-PET can distinguish inflamed from non-inflamed plaque in an animal model of atherosclerosis. Int J Cardiovasc Imaging. 2010; 26:41–48.
28. Rudd JH, Warburton EA, Fryer TD, Jones HA, Clark JC, Antoun N, et al. Imaging atherosclerotic plaque inflammation with [18F]-fluorodeoxyglucose positron emission tomography. Circulation. 2002; 105:2708–2711.
29. Tawakol A, Migrino RQ, Bashian GG, Bedri S, Vermylen D, Cury RC, et al. In vivo 18F-fluorodeoxyglucose positron emission tomography imaging provides a noninvasive measure of carotid plaque inflammation in patients. J Am Coll Cardiol. 2006; 48:1818–1824.
30. Graebe M, Pedersen SF, Borgwardt L, Højgaard L, Sillesen H, Kjaer A. Molecular pathology in vulnerable carotid plaques: correlation with [18]-fluorodeoxyglucose positron emission tomography (FDG-PET). Eur J Vasc Endovasc Surg. 2009; 37:714–721.
31. Silvera SS, Aidi HE, Rudd JH, Mani V, Yang L, Farkouh M, et al. Multimodality imaging of atherosclerotic plaque activity and composition using FDG-PET/CT and MRI in carotid and femoral arteries. Atherosclerosis. 2009; 207:139–143.
32. Wu YW, Kao HL, Chen MF, Lee BC, Tseng WY, Jeng JS, et al. Characterization of plaques using 18F-FDG PET/CT in patients with carotid atherosclerosis and correlation with matrix metalloproteinase-1. J Nucl Med. 2007; 48:227–233.
33. Tahara N, Kai H, Yamagishi S, Mizoguchi M, Nakaura H, Ishibashi M, et al. Vascular inflammation evaluated by [18F]-fluorodeoxyglucose positron emission tomography is associated with the metabolic syndrome. J Am Coll Cardiol. 2007; 49:1533–1539.
34. Lee DH, Lee SJ, Lee DJ, Kwon SH, Jo KS, An YS, et al. Carotid Artery FDG Uptake May Serve as a Biomarker for Cardiovascular Risk Stratification in Asymptomatic Adults. Nucl Med Mol Imaging. 2014; 48:196–202.
35. Wassélius J, Larsson S, Sundin A, Jacobsson H. Assessment of inactive, active and mixed atherosclerotic plaques by 18F-FDG-PET; an age group-based correlation with cardiovascular risk factors. Int J Cardiovasc Imaging. 2009; 25:133–140.
36. Bural GG, Torigian DA, Chamroonrat W, Houseni M, Chen W, Basu S, et al. FDG-PET is an effective imaging modality to detect and quantify age-related atherosclerosis in large arteries. Eur J Nucl Med Mol Imaging. 2008; 35:562–569.
37. Ben-Haim S, Kupzov E, Tamir A, Frenkel A, Israel O. Changing patterns of abnormal vascular wall F-18 fluorodeoxyglucose uptake on follow-up PET/CT studies. J Nucl Cardiol. 2006; 13:791–800.
38. Rudd JH, Myers KS, Bansilal S, Machac J, Woodward M, Fuster V, et al. Relationships among regional arterial inflammation, calcification, risk factors, and biomarkers: a prospective fluorodeoxyglucose positron-emission tomography/computed tomography imaging study. Circ Cardiovasc Imaging. 2009; 2:107–115.
39. Wassélius JA, Larsson SA, Jacobsson H. FDG-accumulating atherosclerotic plaques identified with 18F-FDG-PET/CT in 141 patients. Mol Imaging Biol. 2009; 11:455–459.
40. Figueroa AL, Abdelbaky A, Truong QA, Corsini E, MacNabb MH, Lavender ZR, et al. Measurement of arterial activity on routine FDG PET/CT images improves prediction of risk of future CV events. JACC Cardiovasc Imaging. 2013; 6:1250–1259.
41. Müller HF, Viaccoz A, Fisch L, Bonvin C, Lovblad KO, Ratib O, et al. 18FDG-PET-CT: an imaging biomarker of high-risk carotid plaques. Correlation to symptoms and microembolic signals. Stroke. 2014; 45:3561–3566.
42. Rominger A, Saam T, Wolpers S, Cyran CC, Schmidt M, Foerster S, et al. 18F-FDG PET/CT identifies patients at risk for future vascular events in an otherwise asymptomatic cohort with neoplastic disease. J Nucl Med. 2009; 50:1611–1620.
43. Tahara N, Kai H, Ishibashi M, Nakaura H, Kaida H, Baba K, et al. Simvastatin attenuates plaque inflammation: evaluation by fluorodeoxyglucose positron emission tomography. J Am Coll Cardiol. 2006; 48:1825–1831.
44. Potter K, Lenzo N, Eikelboom JW, Arnolda LF, Beer C, Hankey GJ. Effect of long-term homocysteine reduction with B vitamins on arterial wall inflammation assessed by fluorodeoxyglucose positron emission tomography: a randomised double-blind, placebo-controlled trial. Cerebrovasc Dis. 2009; 27:259–265.
45. Jeong J, Kong E, Chun K, Cho I. The Impact of Energy Substrates, Hormone Level and Subject-Related Factors on Physiologic Myocardial (18)F-FDG Uptake in Normal Humans. Nucl Med Mol Imaging. 2013; 47:225–231.
46. Morooka M, Moroi M, Uno K, Ito K, Wu J, Nakagawa T, et al. Long fasting is effective in inhibiting physiological myocardial 18F-FDG uptake and for evaluating active lesions of cardiac sarcoidosis. EJNMMI Res. 2014; 4:1.
47. Gormsen LC, Christensen NL, Bendstrup E, Tolbod LP, Nielsen SS. Complete somatostatin-induced insulin suppression combined with heparin loading does not significantly suppress myocardial 18F-FDG uptake in patients with suspected cardiac sarcoidosis. J Nucl Cardiol. 2013; 20:1108–1115.
48. Harisankar CN, Mittal BR, Agrawal KL, Abrar ML, Bhattacharya A. Utility of high fat and low carbohydrate diet in suppressing myocardial FDG uptake. J Nucl Cardiol. 2011; 18:926–936.
49. Annovazzi A, Bonanno E, Arca M, D'Alessandria C, Marcoccia A, Spagnoli LG, et al. 99mTc-interleukin-2 scintigraphy for the in vivo imaging of vulnerable atherosclerotic plaques. Eur J Nucl Med Mol Imaging. 2006; 33:117–126.
50. Elmaleh DR, Narula J, Babich JW, Petrov A, Fischman AJ, Khaw BA, et al. Rapid noninvasive detection of experimental atherosclerotic lesions with novel 99mTc-labeled diadenosine tetraphosphates. Proc Natl Acad Sci U S A. 1998; 95:691–695.
51. Tepe G, Duda SH, Meding J, Brehme U, Ritter J, Hanke H, et al. Tc-99m-labeled endothelin derivative for imaging of experimentally induced atherosclerosis. Atherosclerosis. 2001; 157:383–392.
52. Glaudemans AW, Bonanno E, Galli F, Zeebregts CJ, de Vries EF, Koole M, et al. In vivo and in vitro evidence that 99mTc-HYNIC-interleukin-2 is able to detect T lymphocytes in vulnerable atherosclerotic plaques of the carotid artery. Eur J Nucl Med Mol Imaging. 2014; 41:1710–1719.
53. Liu Y, Pierce R, Luehmann HP, Sharp TL, Welch MJ. PET imaging of chemokine receptors in vascular injury-accelerated atherosclerosis. J Nucl Med. 2013; 54:1135–1141.
54. Luehmann HP, Pressly ED, Detering L, Wang C, Pierce R, Woodard PK, et al. PET/CT imaging of chemokine receptor CCR5 in vascular injury model using targeted nanoparticle. J Nucl Med. 2014; 55:629–634.
55. Winkel LC, Groen HC, van Thiel BS, Müller C, van der Steen AF, Wentzel JJ, et al. Folate receptor-targeted single-photon emission computed tomography/computed tomography to detect activated macrophages in atherosclerosis: can it distinguish vulnerable from stable atherosclerotic plaques? Mol Imaging. 2014; 13.
56. Jager NA, Westra J, Golestani R, van Dam GM, Low PS, Tio RA, et al. Folate receptor-β imaging using 99mTc-folate to explore distribution of polarized macrophage populations in human atherosclerotic plaque. J Nucl Med. 2014; 55:1945–1951.
57. Tahara N, Mukherjee J, de Haas HJ, Petrov AD, Tawakol A, Haider N, et al. 2-deoxy-2-[18F]fluoro-D-mannose positron emission tomography imaging in atherosclerosis. Nat Med. 2014; 20:215–219.
58. Li D, Patel AR, Klibanov AL, Kramer CM, Ruiz M, Kang BY, et al. Molecular imaging of atherosclerotic plaques targeted to oxidized LDL receptor LOX-1 by SPECT/CT and magnetic resonance. Circ Cardiovasc Imaging. 2010; 3:464–472.
59. Rominger A, Saam T, Vogl E, Ubleis C, la Fougère C, Förster S, et al. In vivo imaging of macrophage activity in the coronary arteries using 68Ga-DOTATATE PET/CT: correlation with coronary calcium burden and risk factors. J Nucl Med. 2010; 51:193–197.
60. Li X, Samnick S, Lapa C, Israel I, Buck AK, Kreissl MC, et al. 68Ga-DOTATATE PET/CT for the detection of inflammation of large arteries: correlation with18F-FDG, calcium burden and risk factors. EJNMMI Res. 2012; 2:52.
61. Mojtahedi A, Alavi A, Thamake S, Amerinia R, Ranganathan D, Tworowska I, et al. Assessment of vulnerable atherosclerotic and fibrotic plaques in coronary arteries using (68)Ga-DOTATATE PET/CT. Am J Nucl Med Mol Imaging. 2014; 5:65–71.
62. Laitinen I, Marjamäki P, Någren K, Laine VJ, Wilson I, Leppänen P, et al. Uptake of inflammatory cell marker [11C]PK11195 into mouse atherosclerotic plaques. Eur J Nucl Med Mol Imaging. 2009; 36:73–80.
63. Bird JL, Izquierdo-Garcia D, Davies JR, Rudd JH, Probst KC, Figg N, et al. Evaluation of translocator protein quantification as a tool for characterising macrophage burden in human carotid atherosclerosis. Atherosclerosis. 2010; 210:388–391.
64. Pugliese F, Gaemperli O, Kinderlerer AR, Lamare F, Shalhoub J, Davies AH, et al. Imaging of vascular inflammation with [11C]-PK11195 and positron emission tomography/computed tomography angiography. J Am Coll Cardiol. 2010; 56:653–661.
65. Gaemperli O, Shalhoub J, Owen DR, Lamare F, Johansson S, Fouladi N, et al. Imaging intraplaque inflammation in carotid atherosclerosis with 11C-PK11195 positron emission tomography/computed tomography. Eur Heart J. 2012; 33:1902–1910.
66. Laitinen IE, Luoto P, Någren K, Marjamäki PM, Silvola JM, Hellberg S, et al. Uptake of 11C-choline in mouse atherosclerotic plaques. J Nucl Med. 2010; 51:798–802.
67. Bucerius J, Schmaljohann J, Böhm I, Palmedo H, Guhlke S, Tiemann K, et al. Feasibility of 18F-fluoromethylcholine PET/CT for imaging of vessel wall alterations in humans--first results. Eur J Nucl Med Mol Imaging. 2008; 35:815–820.
68. Kato K, Schober O, Ikeda M, Schäfers M, Ishigaki T, Kies P, et al. Evaluation and comparison of 11C-choline uptake and calcification in aortic and common carotid arterial walls with combined PET/CT. Eur J Nucl Med Mol Imaging. 2009; 36:1622–1628.
69. Nahrendorf M, Zhang H, Hembrador S, Panizzi P, Sosnovik DE, Aikawa E, et al. Nanoparticle PET-CT imaging of macrophages in inflammatory atherosclerosis. Circulation. 2008; 117:379–387.
70. Schäfers M, Riemann B, Kopka K, Breyholz HJ, Wagner S, Schäfers KP, et al. Scintigraphic imaging of matrix metalloproteinase activity in the arterial wall in vivo. Circulation. 2004; 109:2554–2559.
71. Fujimoto S, Hartung D, Ohshima S, Edwards DS, Zhou J, Yalamanchili P, et al. Molecular imaging of matrix metalloproteinase in atherosclerotic lesions: resolution with dietary modification and statin therapy. J Am Coll Cardiol. 2008; 52:1847–1857.
72. Breyholz HJ, Wagner S, Levkau B, Schober O, Schäfers M, Kopka K. A 18F-radiolabeled analogue of CGS 27023A as a potential agent for assessment of matrix-metalloproteinase activity in vivo. Q J Nucl Med Mol Imaging. 2007; 51:24–32.
73. Tavakoli S, Razavian M, Zhang J, Nie L, Marfatia R, Dobrucki LW, et al. Matrix metalloproteinase activation predicts amelioration of remodeling after dietary modification in injured arteries. Arterioscler Thromb Vasc Biol. 2011; 31:102–109.
74. Razavian M, Tavakoli S, Zhang J, Nie L, Dobrucki LW, Sinusas AJ, et al. Atherosclerosis plaque heterogeneity and response to therapy detected by in vivo molecular imaging of matrix metalloproteinase activation. J Nucl Med. 2011; 52:1795–1802.
75. Weis SM, Cheresh DA. Tumor angiogenesis: molecular pathways and therapeutic targets. Nat Med. 2011; 17:1359–1370.
76. Razavian M, Marfatia R, Mongue-Din H, Tavakoli S, Sinusas AJ, Zhang J, et al. Integrin-targeted imaging of inflammation in vascular remodeling. Arterioscler Thromb Vasc Biol. 2011; 31:2820–2826.
77. Paeng JC, Lee YS, Lee JS, Jeong JM, Kim KB, Chung JK, et al. Feasibility and kinetic characteristics of (68)Ga-NOTA-RGD PET for in vivo atherosclerosis imaging. Ann Nucl Med. 2013; 27:847–854.
78. Su H, Gorodny N, Gomez LF, Gangadharmath UB, Mu F, Chen G, et al. Atherosclerotic plaque uptake of a novel integrin tracer 18F-Flotegatide in a mouse model of atherosclerosis. J Nucl Cardiol. 2014; 21:553–562.
79. Beer AJ, Pelisek J, Heider P, Saraste A, Reeps C, Metz S, et al. PET/CT imaging of integrin αvβ3 expression in human carotid atherosclerosis. JACC Cardiovasc Imaging. 2014; 7:178–187.
80. Laitinen I, Saraste A, Weidl E, Poethko T, Weber AW, Nekolla SG, et al. Evaluation of alphavbeta3 integrin-targeted positron emission tomography tracer 18F-galacto-RGD for imaging of vascular inflammation in atherosclerotic mice. Circ Cardiovasc Imaging. 2009; 2:331–338.
81. Kim YI, Phi JH, Paeng JC, Choi H, Kim SK, Lee YS, et al. In vivo evaluation of angiogenic activity and its correlation with efficacy of indirect revascularization surgery in pediatric moyamoya disease. J Nucl Med. 2014; 55:1467–1472.
82. Golestani R, Zeebregts CJ, Terwisscha van Scheltinga AG, Lub-de Hooge MN, van Dam GM, Glaudemans AW, et al. Feasibility of vascular endothelial growth factor imaging in human atherosclerotic plaque using (89)Zr-bevacizumab positron emission tomography. Mol Imaging. 2013; 12:235–243.
83. Krohn KA, Link JM, Mason RP. Molecular imaging of hypoxia. J Nucl Med. 2008; 49:Suppl 2. 129S–148S.
84. Mateo J, Izquierdo-Garcia D, Badimon JJ, Fayad ZA, Fuster V. Noninvasive assessment of hypoxia in rabbit advanced atherosclerosis using 18F-fluoromisonidazole positron emission tomographic imaging. Circ Cardiovasc Imaging. 2014; 7:312–320.
85. Kolodgie FD, Petrov A, Virmani R, Narula N, Verjans JW, Weber DK, et al. Targeting of apoptotic macrophages and experimental atheroma with radiolabeled annexin V: a technique with potential for noninvasive imaging of vulnerable plaque. Circulation. 2003; 108:3134–3139.
86. Johnson LL, Schofield L, Donahay T, Narula N, Narula J. 99mTc-annexin V imaging for in vivo detection of atherosclerotic lesions in porcine coronary arteries. J Nucl Med. 2005; 46:1186–1193.
87. Isobe S, Tsimikas S, Zhou J, Fujimoto S, Sarai M, Branks MJ, et al. Noninvasive imaging of atherosclerotic lesions in apolipoprotein E-deficient and low-density-lipoprotein receptor-deficient mice with annexin A5. J Nucl Med. 2006; 47:1497–1505.
88. Dweck MR, Chow MW, Joshi NV, Williams MC, Jones C, Fletcher AM, et al. Coronary arterial 18F-sodium fluoride uptake: a novel marker of plaque biology. J Am Coll Cardiol. 2012; 59:1539–1548.
89. Joshi NV, Vesey AT, Williams MC, Shah AS, Calvert PA, Craighead FH, et al. 18F-fluoride positron emission tomography for identification of ruptured and high-risk coronary atherosclerotic plaques: a prospective clinical trial. Lancet. 2014; 383:705–713.
90. Derlin T, Richter U, Bannas P, Begemann P, Buchert R, Mester J, et al. Feasibility of 18F-sodium fluoride PET/CT for imaging of atherosclerotic plaque. J Nucl Med. 2010; 51:862–865.
91. Derlin T, Tóth Z, Papp L, Wisotzki C, Apostolova I, Habermann CR, et al. Correlation of inflammation assessed by 18F-FDG PET, active mineral deposition assessed by 18F-fluoride PET, and vascular calcification in atherosclerotic plaque: a dual-tracer PET/CT study. J Nucl Med. 2011; 52:1020–1027.
92. Derlin T, Wisotzki C, Richter U, Apostolova I, Bannas P, Weber C, et al. In vivo imaging of mineral deposition in carotid plaque using 18F-sodium fluoride PET/CT: correlation with atherogenic risk factors. J Nucl Med. 2011; 52:362–368.
93. Li Y, Berenji GR, Shaba WF, Tafti B, Yevdayev E, Dadparvar S. Association of vascular fluoride uptake with vascular calcification and coronary artery disease. Nucl Med Commun. 2012; 33:14–20.
94. Rudd JH, Myers KS, Bansilal S, Machac J, Rafique A, Farkouh M, et al. (18)Fluorodeoxyglucose positron emission tomography imaging of atherosclerotic plaque inflammation is highly reproducible: implications for atherosclerosis therapy trials. J Am Coll Cardiol. 2007; 50:892–896.
95. Rudd JH, Myers KS, Bansilal S, Machac J, Pinto CA, Tong C, et al. Atherosclerosis inflammation imaging with 18F-FDG PET: carotid, iliac, and femoral uptake reproducibility, quantification methods, and recommendations. J Nucl Med. 2008; 49:871–878.
96. Wassélius J, Larsson S, Jacobsson H. Time-to-time correlation of high-risk atherosclerotic lesions identified with [(18)F]-FDG-PET/CT. Ann Nucl Med. 2009; 23:59–64.