Abstract
Hyperglycemia is associated with an increased risk of cardiovascular diseases. It has been demonstrated that chronic exposure to high glucose impaired endothelial functions. However, specific effects of short-term exposure to high glucose on vascular reactivity are controversial. Moreover, the combined effects of other metabolic substrates such as free fatty acids (FFA) on vascular reactivity remain poorly understood. Here we investigate the effects of short-term exposure to high glucose with or without other metabolic substrates including FFAs termed “nutrition full” (NF) solution, on mesenteric (MA) and deep femoral arteries (DFA) of rats. Arterial ring segments were mounted in a double-wire myograph. Contraction in response to phenylephrine (PhE) was determined in control (5 mM) and high glucose (23 mM, HG) environments over a 30 min period. In both arteries, PhE-inducedvasocontraction was enhanced by pre-incubation of HG solution. A combined incubation with HG and palmitic acid (100 µM) induced similar sensitization of PhE-contractions in both arteries. In contrast, high K+-induced contractions were not affected by HG. Interestingly, pre-incubation with NF solution decreased PhE-induced contraction in MA but increased the contraction in DFA. In NF solution, the HG-induced facilitation of PhE-contraction was not observed in MA. Furthermore, the PhE-induced contraction of DFA was attenuated by HG in NF solution. Our results demonstrate that the sensitization of PhE-induced arterial contraction by HG is differentially affected by other metabolic substrates. The conversation of skeletal arterial contractility by HG in NF solution requires careful interpretation of the previous in vitro studies where only glucose is included in physiological salt solutions. Further studies are required to elucidate the mechanism underlying the inconsistent effect of NF solution on MA and DFA.
Peripheral arterial disease is a common complication in patients with diabetes [1]. Hyperglycemia causes vascular damage with several underlying mechanisms. Among them, high glucose levels in the blood may contribute to endothelial dysfunction by mediating abnormal endothelial permeability or an imbalance between endothelium-derived relaxing and contracting factors [2345678]. Several previous studies also demonstrated that prolonged exposure to high glucose may affect endothelium-dependent vasodilation by stimulating the production of reactive oxygen species (ROS) [9101112] or voltage-gated K+ channel function in coronary vascular smooth muscle cells [13]. The majority of previous studies demonstrated effects of hyperglycemia on vascular functions with long-term exposure; however, a few studies have been conducted to determine the effects of high glucose with short-term exposure. The results of those studies varied with the different experimental conditions [141516]. The incubation of high glucose (20 mM) for 2 h induced impairment of bradykinin-induced relaxation in human subcutaneous arteries whereas induced opposite result in human mesenteric arteries (MAs) [15]. Also, exposure of high glucose (44 mM) for 3h produced the attenuation of phenylephrine (PhE)-induced contraction in rat aortic rings [14].
Free fatty acids (FFAs) regulate vascular functions, and circulating FFA levels in blood serum are often associated with hyperglycemia. Some studies have demonstrated that excessive levels FFAs may cause vascular dysfunction by enhancing oxidative stress or reducing nitric oxide (NO) production in endothelial cells [17181920]. A previous study has suggested that exposure to FFAs at both high and physiological concentrations impaired endothelium-dependent relaxation (EDR) in MA [21]. However, results from another study showed that FFAs did not affect endothelial function or ROS generation in the vessels [20].
Conventional in vitro studies of arterial contractility is conducted in physiological salt solutions with glucose only for the metabolic substrates. Considering the importance of vascular functions in the pathophysiology of metabolic diseases, it is requested to have a comprehensive understanding of systemic arterial contractility under physiological conditions. On these backgrounds, we investigate the effects of short-term incubation with high glucose with or without other metabolic substrates on α-adrenergic agonist-induced contraction in rat mesenteric arteries (MAs) and deep femoral arteries (DFAs).
Experiments were performed with the approval of the Institutional Animal Care and Use Committee in Chung-Ang University (approval no. 2015-00012). Adult male Sprague-Dawley rats (240~320 g) were anesthetized by intraperitoneal injection of pentobarbital sodium (60~100 mg/kg). Full anesthesia was confirmed by the absence of limb withdrawal using toe pinching. The intestine and proximal hind limbs were removed quickly and placed into normal Tyrode (NT) solution at 4℃. The NT solution contained the following (in mM): NaCl 140, KCl 5.4, 4-(2-hydroxyethyl)-1-piperazineethanesulfonic acid 10, NaH2PO4 0.33, glucose 10, CaCl2 1.8, and MgCl2 1. The NT solution was adjusted to pH 7.4 with NaOH. Arteries were dissected from fat and connective tissue in NT solution. The second and third branches of MAs and DFAs were isolated quickly (inner diameter: 200~300 µm) and cut into segments 2.5~3 mm in length.
Endothelium-intact artery rings were mounted on two 25 µm tungsten wires in a dual-wire myograph (620 M; DMT, Aarhus, Denmark). Each myograph chamber was filled with 5 mL of physiological salt solution (PSS). An arterial ring segment was stabilized in PSS aerated with 21% O2,/5% CO2 (the remainder N2) at 37℃. The PSS solution consisted of the following (in mM): NaCl 118, KCl 4, MgSO4 1, NaHCO3 24, NaH2PO4 0.44, glucose 5.6, and CaCl2 1.8.
For each vessel, 80 mM KCl-PSS was used to obtain initial maximal contractility. To compare between groups, the contractile responses induced by different concentrations of phenylephrine (PhE) were normalized to the 80 mM KCl-induced maximum constriction in each vessel. In addition, EDR was measured by the response to 10 µM acetylcholine (ACh) with rings pre-contracted with 10 µM PhE. The contractile response induced by PhE or KCl was assessed in the presence or absence of high glucose (23 mM), or with metabolic substrates.
Metabolic substrates were added to the PSS solution. The metabolic substrate compositions were modified based on analyses of the human's blood sample [22]. The NF solution consisted of the following (in mM): 96 mL PSS, oleic acid 200 µM, palmitic acid 100 µM, linoleic acid 100 µM, sodium L-lactate 1 mM, sodium pyruvate 100 µM, and carnitine hydrochloride 50 µM. All fatty acids were prepared at physiological concentrations. Palmitic acid, linoleic acid, and oleic acid were dissolved in 0.1 N NaOH. All drugs and chemicals were purchased from Sigma-Aldrich (St. Louis, MO, USA).
Data are shown as means±SEM. The response to PhE is expressed as the percent of the second 80 mM KCl-PSS-induced maximum constriction. Half-maximal effective concentrations (EC50 levels) were calculated by non-linear regression analyses of the concentration response curves generated with OriginPro 8.0 software (OriginLab, Northampton, MA, USA). Statistical analysis was performed with the unpaired Student's t-test. Statistical significance is reported as p<0.05.
After confirming 80 mM KCl-induced (80K contraction) and 10 µM PhE-induced contraction, endothelium-dependent ACh-induced vasorelaxation was tested in each vessel. The tested vessels were either incubated with 23 mM glucose-containing or control PSS, and then the concentration-dependent contractile responses to PhE were analyzed. Incremental application of PhE (0.05~10 µM) induced stepwise increase of arterial tones in both MAs and DFAs (Fig. 1). Noticeable contraction was observed from 1 µM PhE. In general, the PhE- contractions at 2 µM and above were more persistent in DFAs than MAs; MAs showed partial relaxation following peak contractions. Thus, for the comparison between MAs and DFAs, the peak amplitudes of PhE- contraction were analyzed after normalization to 80 K contraction in each vessel. After 30 min incubation in the presence of high glucose (23 mM, HG), the amplitudes of PhE-contraction became higher in both MAs and DFAs (Fig. 1). The EC50 values of PhE-contraction in MAs were 1.96±0.37 and 1.22±0.25 µM for control and HG, respectively (Fig. 1A), and 2.41±0.14 and 1.61±0.34 µM for control and HG in DFAs (Fig. 1B), respectively.
To determine the effect of HG on the purely membrane depolarization-dependent contraction, cumulative doses of KCl were applied to both arteries (Fig. 2). Contractile responses to KCl were unaffected in the presence of HG (Fig. 2C, F).
Next, we tested whether combined application of HG and saturated fatty acid differently affects the PhE- contractions. When co-incubated with palmitic acid (100 µM), the enhancement of PhE-contraction by HG was similarly observed in MAs and DFAs (Fig. 3). The EC50 values were: control MAs, 1.96±0.27 µM; high glucose MAs, 1.41±0.33 µM; control DFAs, 2.41±0.19 µM; high glucose DFAs, 1.20±0.05 µM. It has to be noted that the concentrations-dependent PhE- contraction data for the control condition were same ones in Fig. 1 and Fig. 3.
Then we tested the effects of NF solution on PhE-contractions. A significant reduction of PhE (1~5 µM)-induced contraction was observed when incubating MA rings with NF solution for 30 min (Fig. 4A, B). EC50 values were: control, 1.96±0.31 µM; NF, 3.08±0.38 µM. Interestingly, however, the incubation of DFA rings with NF solution for 30 min produced an increased sensitivity to PhE-induced contraction (Fig. 4). The NF solution caused a significant leftward shift of the PhE (1 and 2 µM) concentration-response curve (Fig. 4C, D). EC50 values were: control, 2.41±0.14 µM; NF, 1.36±0.22 µM.
The co-incubation with HG in NF solutions did not increase but slightly decreased the sensitivity to PhE- contraction in MAs (Fig. 5A, B). EC50 values were 1.96±0.28 and 3.07±0.27 µM for control and HG with NF in MAs, respectively (Fig. 5B). More interestingly, the enhanced PhE- contraction of DFAs under NF solution became reversed to inhibition smaller than the control level. Significant rightward shifts of the concentration-response curves to PhE (1 and 2 µM in MAs, 1 and 10 µM in DFAs) were observed in the presence of the combined high glucose and NF solutions (Fig. 5B, D). EC50 value was 2.99±0.18 µM for HG/NF in DFAs (Fig. 5D), respectively.
The results of the present study showed that only 30 min of incubation in HG enhances the sensitivity to PhE-induced contraction in rat MAs and DFAs. More interesting finding was that the incubation in NF solution induces opposite responses, decrease and increase of PhE-contractions in MAs and DFAs, respectively. Furthermore, the pro-contractile effect of HG alone converts to an attenuation of PhE-contraction in NF solution, especially in DFA.
The effects of HG on PhE-induced contraction vary depending on the experimental conditions [1415162324]. Although long term exposure to high glucose inhibits EDR in a majority of studies [252627], several studies show conflicting results with regard to the effects of short-term exposure to high glucose [14151628]. A previous study using diabetic rats showed decreased sensitivity to PhE-induced contraction in blood vessels [24] whereas another study demonstrated the opposite result [29]. A more recent study also reported that PhE-induced contraction in aortic rings was attenuated by acute exposure to high glucose (44 mM) for 3 h [14].
In the conventional PSS solution, the acute exposure to HG consistently enhanced the PhE-contraction in both MAs and DFAs (Fig. 1). Because the high K+-induced contractions were not affected by HG, the procontractile effects appears to be more specifically associated with the a-adrenergic signaling pathways or might be mediated by K+ channel inhibition [13]. The absence of any change in the high-K+ contraction also suggests that the endothelial function is not acutely changed by HG for 30 min. This supposition is supported by a previous finding that the relaxation produced by ACh was induced by a 6 but not 2 h incubation in high glucose (20 mM) in human mesenteric and subcutaneous arteries [15].
However, we could not exclude the effects of acute HG on endothelial functions. Several studies demonstrated that impairment of EDR augmented PhE-induced contraction following prolonged exposure to high glucose [5]. In addition, a high concentration of glucose induced endothelial dysfunction as shown by decreased ACh-induced NO-mediated relaxation [7830] and increased production of ROS in cultured endothelial cells [718]. NADPH oxidase (NOX) is one of the most important sources of ROS [31]. Several studies have indicated that high glucose activates NOX and induces ROS production in endothelial and vascular smooth muscle cells via a protein kinase C-dependent pathway [3233], and that ROS interact with endothelial NO to reduce NO bioactivity [34]. Therefore, a decrease of EDR by short-term exposure to high glucose could affect increased sensitivity to PhE-induced contraction in MAs and DFAs.
The glucose concentration we applied in the present study was similar to postprandial glucose levels in Type II diabetic patients [35]. Initially, it was suggested that the augmentation of PhE-contraction by the acute HG suggested that the total peripheral resistance under tonic sympathetic tone might induce an increase of blood pressure after some heavy meals. However, the differential effects of HG on PhE-contractions in NF solution imply that the actual influence of glucose uptake on the resistance of circulation system requires careful interpretation.
In the present study, the effects of NF solution were demonstrated in rat blood vessels (Fig. 4). In rat MAs and DFAs, the application of NF solutions showed inconsistent effects on PhE-induced contraction. The mechanism underlying this inconsistency is unclear. However, we cautiously assume that the specific composition of the NF solution may reduce sensitivity to PhE-induced contraction in MAs. At first, we assumed that saturated fatty acids such as palmitic acid might be responsible for the differential responses in NF. However, it was found that the sensitivity to PhE-induced contraction was consistently augmented by co-incubation with palmitic acid and high glucose in both arteries (Fig. 3). Thus, other components of NF, or more complicated effects of the combined components seem to be responsible for the intriguing influence of NF solution.
Previous studies showed that individual free fatty acids have similar effects in MAs and DFAs, and endothelial cell dysfunction was induced with high and physiological concentrations of both saturated and unsaturated fatty acids [2136]. Further investigation is needed to identify these inconsistent effects of NF solutions using various arteries and solution compositions.
In conclusion, the results of the current study demonstrated that short-term exposure to a high glucose solution augmented sensitivity to PhE-induced contraction in rat MAs and DFAs. In addition, this is the first study to examine the effects of NF solution alone and with high glucose. These findings provide preliminary evidence for identifying the action mechanism of metabolic substrates and high glucose on vascular reactivity.
ACKNOWLEDGEMENTS
This study was supported by a National Research Foundation of Korea grant funded by the Ministry of Science, ICT, & Future Planning (2015R1C1A1A01054038).
Notes
References
1. Fowler MJ. Microvascular and macrovascular complications of diabetes. Clinical Diabetes. 2008; 26:77–82.


2. Triggle CR, Samuel SM, Ravishankar S, Marei I, Arunachalam G, Ding H. The endothelium: influencing vascular smooth muscle in many ways. Can J Physiol Pharmacol. 2012; 90:713–738. PMID: 22625870.


3. Sandoo A, van Zanten JJ, Metsios GS, Carroll D, Kitas GD. The endothelium and its role in regulating vascular tone. Open Cardiovasc Med J. 2010; 4:302–312. PMID: 21339899.


4. Rajendran P, Rengarajan T, Thangavel J, Nishigaki Y, Sakthisekaran D, Sethi G, Nishigaki I. The vascular endothelium and human diseases. Int J Biol Sci. 2013; 9:1057–1069. PMID: 24250251.


5. Taylor PD, Poston L. The effect of hyperglycaemia on function of rat isolated mesenteric resistance artery. Br J Pharmacol. 1994; 113:801–808. PMID: 7858870.


6. Akbari CM, Saouaf R, Barnhill DF, Newman PA, LoGerfo FW, Veves A. Endothelium-dependent vasodilatation is impaired in both microcirculation and macrocirculation during acute hyperglycemia. J Vasc Surg. 1998; 28:687–694. PMID: 9786265.


7. Brouwers O, Niessen PM, Haenen G, Miyata T, Brownlee M, Stehouwer CD, De Mey JG, Schalkwijk CG. Hyperglycaemia-induced impairment of endothelium-dependent vasorelaxation in rat mesenteric arteries is mediated by intracellular methylglyoxal levels in a pathway dependent on oxidative stress. Diabetologia. 2010; 53:989–1000. PMID: 20186387.


8. Zhou ZW, Xie XL, Zhou SF, Li CG. Mechanism of reversal of high glucose-induced endothelial nitric oxide synthase uncoupling by tanshinone IIA in human endothelial cell line EA.hy926. Eur J Pharmacol. 2012; 697:97–105. PMID: 23063542.


9. Koo JR, Ni Z, Oviesi F, Vaziri ND. Antioxidant therapy potentiates antihypertensive action of insulin in diabetic rats. Clin Exp Hypertens. 2002; 24:333–344. PMID: 12109774.


10. Marfella R, Quagliaro L, Nappo F, Ceriello A, Giugliano D. Acute hyperglycemia induces an oxidative stress in healthy subjects. J Clin Invest. 2001; 108:635–636. PMID: 11518739.


11. Li PA, Liu GJ, He QP, Floyd RA, Siesjö BK. Production of hydroxyl free radical by brain tissues in hyperglycemic rats subjected to transient forebrain ischemia. Free Radic Biol Med. 1999; 27:1033–1040. PMID: 10569636.


12. Hsieh TJ, Zhang SL, Filep JG, Tang SS, Ingelfinger JR, Chan JS. High glucose stimulates angiotensinogen gene expression via reactive oxygen species generation in rat kidney proximal tubular cells. Endocrinology. 2002; 143:2975–2985. PMID: 12130563.


13. Li H, Chai Q, Gutterman DD, Liu Y. Elevated glucose impairs cAMP-mediated dilation by reducing Kv channel activity in rat small coronary smooth muscle cells. Am J Physiol Heart Circ Physiol. 2003; 285:H1213–H1219. PMID: 12763748.
14. El-Awady MS, El-Agamy DS, Suddek GM, Nader MA. Propolis protects against high glucose-induced vascular endothelial dysfunction in isolated rat aorta. J Physiol Biochem. 2014; 70:247–254. PMID: 24234058.


15. MacKenzie A, Cooper EJ, Dowell FJ. Differential effects of glucose on agonist-induced relaxations in human mesenteric and subcutaneous arteries. Br J Pharmacol. 2008; 153:480–487. PMID: 18037911.


16. Williams SB, Goldfine AB, Timimi FK, Ting HH, Roddy MA, Simonson DC, Creager MA. Acute hyperglycemia attenuates endothelium-dependent vasodilation in humans in vivo. Circulation. 1998; 97:1695–1701. PMID: 9591763.


17. Chinen I, Shimabukuro M, Yamakawa K, Higa N, Matsuzaki T, Noguchi K, Ueda S, Sakanashi M, Takasu N. Vascular lipotoxicity: endothelial dysfunction via fatty-acid-induced reactive oxygen species overproduction in obese Zucker diabetic fatty rats. Endocrinology. 2007; 148:160–165. PMID: 17023526.


18. Nishikawa T, Edelstein D, Du XL, Yamagishi S, Matsumura T, Kaneda Y, Yorek MA, Beebe D, Oates PJ, Hammes HP, Giardino I, Brownlee M. Normalizing mitochondrial superoxide production blocks three pathways of hyperglycaemic damage. Nature. 2000; 404:787–790. PMID: 10783895.


19. Saraswathi V, Wu G, Toborek M, Hennig B. Linoleic acid-induced endothelial activation: role of calcium and peroxynitrite signaling. J Lipid Res. 2004; 45:794–804. PMID: 14993245.
20. Jaimes EA, Hua P, Tian RX, Raij L. Human glomerular endothelium: interplay among glucose, free fatty acids, angiotensin II, and oxidative stress. Am J Physiol Renal Physiol. 2010; 298:F125–F132. PMID: 19864304.


21. Sainsbury CA, Sattar N, Connell JM, Hillier C, Petrie JR. Non-esterified fatty acids impair endothelium-dependent vasodilation in rat mesenteric resistance vessels. Clin Sci (Lond). 2004; 107:625–629. PMID: 15367101.


22. Bhagavan NV, Ha JS, Park JH, Honda SA, Rios CN, Sugiyama C, Fujitani GK, Takeuchi IK, Ha CE. Utility of serum Fatty Acid concentrations as a marker for acute myocardial infarction and their potential role in the formation of ischemia-modified albumin: a pilot study. Clin Chem. 2009; 55:1588–1590. PMID: 19498051.


23. Banskota AH, Tezuka Y, Kadota S. Recent progress in pharmacological research of propolis. Phytother Res. 2001; 15:561–571. PMID: 11746834.


24. Qian L, Wang H, Xia Q, Bruce I, Huang H. Interleukin-2 improves vascular functions in streptozotocin-induced diabetic rats. In : 2005 IEEE Engineering in Medicine and Biology 27th Annual Conference; 2005 17-18 Jan; 2006.
25. Paneni F, Beckman JA, Creager MA, Cosentino F. Diabetes and vascular disease: pathophysiology, clinical consequences, and medical therapy: part I. Eur Heart J. 2013; 34:2436–2443. PMID: 23641007.


26. Gerich JE. Clinical significance, pathogenesis, and management of postprandial hyperglycemia. Arch Intern Med. 2003; 163:1306–1316. PMID: 12796066.


27. Endemann DH, Schiffrin EL. Endothelial dysfunction. J Am Soc Nephrol. 2004; 15:1983–1992. PMID: 15284284.


28. Beckman JA, Goldfine AB, Gordon MB, Creager MA. Ascorbate restores endothelium-dependent vasodilation impaired by acute hyperglycemia in humans. Circulation. 2001; 103:1618–1623. PMID: 11273987.


29. Ahmad M, Turkseven S, Mingone CJ, Gupte SA, Wolin MS, Abraham NG. Heme oxygenase-1 gene expression increases vascular relaxation and decreases inducible nitric oxide synthase in diabetic rats. Cell Mol Biol (Noisy-le-grand). 2005; 51:371–376. PMID: 16309587.
30. Ozkan MH, Uma S. Inhibition of acetylcholine-induced EDHF response by elevated glucose in rat mesenteric artery. Life Sci. 2005; 78:14–21. PMID: 16125203.
31. Rajagopalan S, Kurz S, Münzel T, Tarpey M, Freeman BA, Griendling KK, Harrison DG. Angiotensin II-mediated hypertension in the rat increases vascular superoxide production via membrane NADH/NADPH oxidase activation. Contribution to alterations of vasomotor tone. J Clin Invest. 1996; 97:1916–1923. PMID: 8621776.


32. Inoguchi T, Li P, Umeda F, Yu HY, Kakimoto M, Imamura M, Aoki T, Etoh T, Hashimoto T, Naruse M, Sano H, Utsumi H, Nawata H. High glucose level and free fatty acid stimulate reactive oxygen species production through protein kinase C--dependent activation of NAD(P)H oxidase in cultured vascular cells. Diabetes. 2000; 49:1939–1945. PMID: 11078463.


33. Inoguchi T, Sonta T, Tsubouchi H, Etoh T, Kakimoto M, Sonoda N, Sato N, Sekiguchi N, Kobayashi K, Sumimoto H, Utsumi H, Nawata H. Protein kinase C-dependent increase in reactive oxygen species (ROS) production in vascular tissues of diabetes: role of vascular NAD(P)H oxidase. J Am Soc Nephrol. 2003; 14(8 Suppl 3):S227–S232. PMID: 12874436.


34. Tomasian D, Keaney JF, Vita JA. Antioxidants and the bioactivity of endothelium-derived nitric oxide. Cardiovasc Res. 2000; 47:426–435. PMID: 10963716.


35. Kipnes M, Dandona P, Tripathy D, Still JG, Kosutic G. Control of postprandial plasma glucose by an oral insulin product (HIM2) in patients with type 2 diabetes. Diabetes Care. 2003; 26:421–426. PMID: 12547873.


36. Lundman P, Tornvall P, Nilsson L, Pernow J. A triglyceride-rich fat emulsion and free fatty acids but not very low density lipoproteins impair endothelium-dependent vasorelaxation. Atherosclerosis. 2001; 159:35–41. PMID: 11689204.


Fig. 1
Effect of high glucose on phenylephrine (PhE)-induced contraction in rat mesenteric arteries (MAs) and deep femoral arteries (DFAs).
Representative traces of PhE-induced contraction in MAs (A, B) and DFAs (D, E) in the absence and presence of high glucose. After incubation in normal (5 mM) or high glucose (23 mM) solution for 30 min, different concentrations of PhE (0.05, 0.1, 1, 2, 5, and 10 µM) were applied. Cumulative log concentration-response curves to PhE in MAs (C) and DFAs (F) in control and high glucose groups are shown as means±SEM. *p<0.05, ***p<0.001.
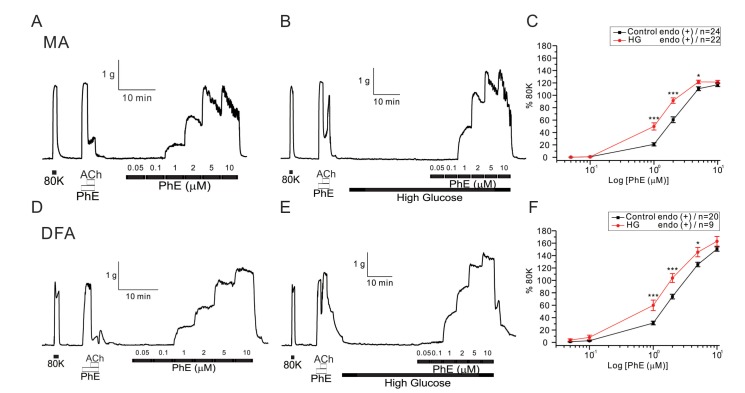
Fig. 2
Effect of high glucose on K+-induced vasoconstriction in rat mesenteric arteries (MAs) and deep femoral arteries (DFAs).
Representative traces of K+-induced vasoconstriction in MA and DFA rings incubated with normal glucose-PSS (A, D) and high glucose-PSS (B, E). Between control and high glucose groups in both arteries, the effect of applying high concentrations of KCl (20 to 120 mM) was not significantly different. Summaries of concentration-response curves for K+-induced vasoconstriction in MAs (C) and DFAs (F) are expressed as means±SEM.
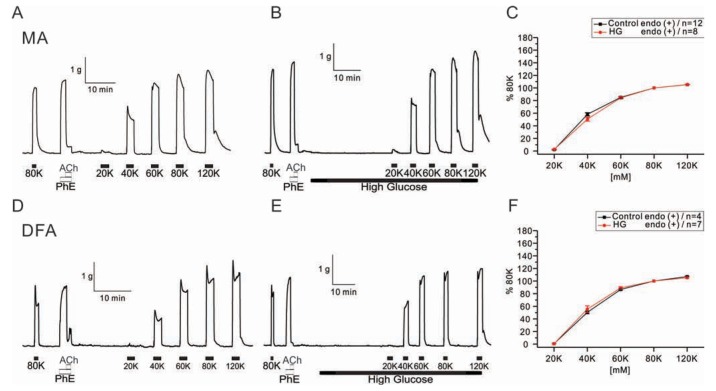
Fig. 3
Effect of co-incubation with high glucose and palmitic acid (HG+PA) solutions on PhE-induced contraction in rat mesenteric arteries (MAs) and deep femoral arteries (DFAs).
Representative traces of isometric tension recordings in HG+PA from MAs (A) and DFAs (C). Summaries of concentration-response curves to PhE in MAs (B) and DFAs (D) are expressed as means ±SEM. **p<0.01, ***p<0.001.
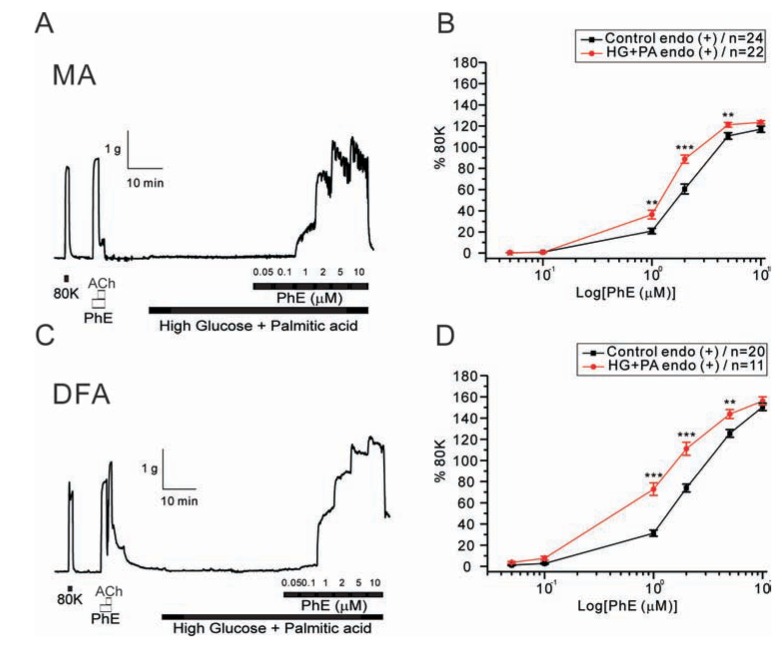
Fig. 4
Effects of NF solutions on PhE-induced contraction in rat mesenteric arteries (MAs) and deep femoral arteries (DFAs).
Representative traces of isometric tension recordings from MAs (A) and DFAs (C). In the absence or presence of NF solutions, different concentrations of PhE (0.05, 0.1, 1, 2, 5, and 10 µM) were applied. PhE-induced contraction was attenuated by NF solutions in MAs whereas contraction was augmented in DFAs. Cumulative log concentration-response curves to PhE in MAs (B) and DFAs (D) in the control and NF groups are expressed as means±SEM. *p<0.05, **p<0.01, ***p<0.001.
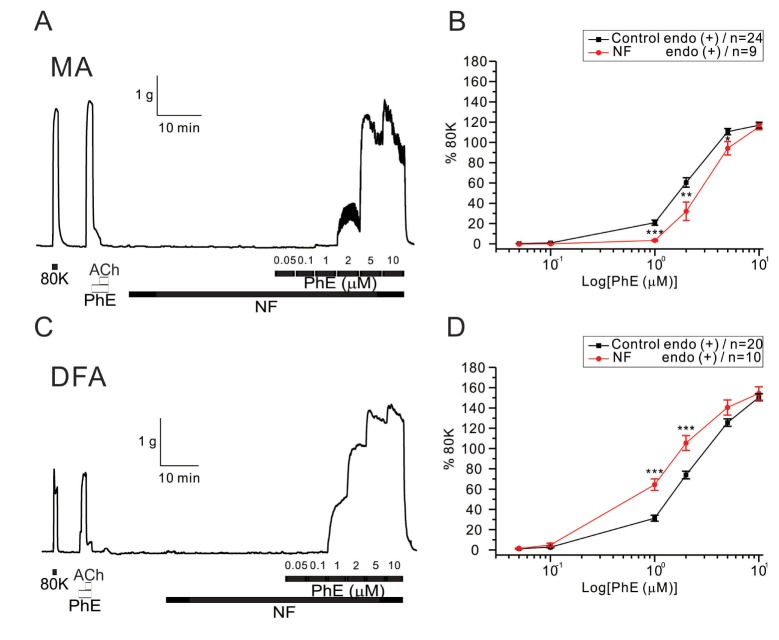
Fig. 5
Effects of co-treatment with high glucose (HG) and NF solutions on PhE-induced contraction in rat mesenteric arteries (MAs) and deep femoral arteries (DFAs).
In both arteries, co-incubation with HG and NF solutions dose-dependently attenuated contractility to PhE (0.05, 0.1, 1, 2, 5, and 10 µM). Data are shown as representative figures of isometric tension recordings in MAs (A) and DFAs (C). Summaries of concentration-response curves to PhE in MAs (B) and DFAs (D) are expressed as means±SEM. *p<0.05, **p<0.01, ***p<0.001.
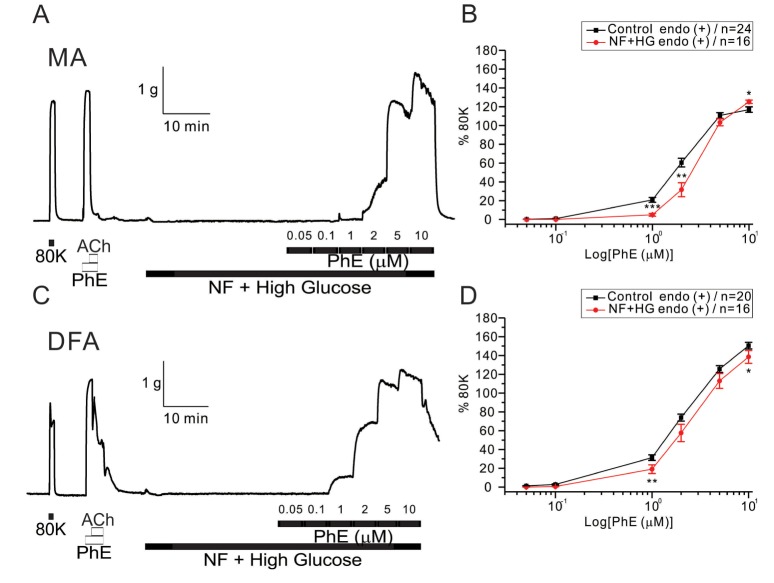