Abstract
Mast cells are primary mediators of allergic inflammation. Beta-1,3-glucan (BG) protects against infection and shock by activating immune cells. Activation of the BG receptor induces an increase in intracellular Ca2+, which may induce exocytosis. However, little is known about the precise mechanisms underlying BG activation of immune cells and the possible role of mitochondria in this process. The present study examined whether BG induced mast cell degranulation, and evaluated the role of calcium transients during mast cell activation. Our investigation focused on the role of the mitochondrial calcium uniporter (MCU) in BG-induced degranulation. Black mouse (C57) bone marrow-derived mast cells were stimulated with 0.5 µg/ml BG, 100 µg/ml peptidoglycan (PGN), or 10 µM A23187 (calcium ionophore), and dynamic changes in cytosolic and mitochondrial calcium and membrane potential were monitored. BG-induced mast cell degranulation occurred in a time-dependent manner, and was significantly reduced under calcium-free conditions. Ruthenium red, a mitochondrial Ca2+ uniporter blocker, significantly reduced mast cell degranulation induced by BG, PGN, and A23187. These results suggest that the mitochondrial Ca2+ uniporter has an important regulatory role in BG-induced mast cell degranulation.
Mast cells are found in several tissues throughout the body, primarily in blood vessels, nerves, and organ systems that interact with the external environment. Bone marrow-derived mast cells express a variety of phenotypic features and are crucial in numerous biological functions [1]. Various stimuli activate mast cells in order to proceed with degranulation, a process during which secretory vesicles release specific secondary metabolites. Mast cell activation can be mediated by pathways that are either dependent or independent on high-affinity IgE receptor (FcεRI). Multivalent antigen (allergen) may interact with its specific IgE antibody attached to the cell membrane via its FcεRI [2]. Mast cells can also be activated by non-immunological substances such as neuropeptides, basic compounds, complement components, and certain drugs [3]. Mast cell degranulation induced by immunological and non-immunological stimuli appear morphologically similar. However, the biochemical processes that lead to mediator release may differ. In FcεRI-dependent pathways, FcεRI phosphorylation induces exocytosis, which increases cytosolic calcium and induces degranulation. The concurrent activation of Ca2+ signaling by inositol-1,4,5-P3 (IP3) and protein kinase C (PKC) signaling by diacylglycerol (DAG) interact synergistically to elicit mast cell degranulation [4]. In FcεRI-independent pathways, mast cells may be activated by numerous stimuli including basic compounds (compound 48/80, mastoparan, and polymyxin B), peptides (melittin, substance P, calcitonin gene-related peptide, somatostatin, and neurotensin), and cytokines (IL-1, IL-3, GM-CSF, platelet factor 4, and IL-8) [1].
Beta-1,3-glucan (BG), which is found in the cell wall of many fungi, has been reported to modulate immunity [5]. It was isolated from S. cerevisiae and was originally reported as zymosan, a kind of β-1,3-glucan, in 1959 by Benacerraf, and demonstrated that it produced hyperplasia and hyper functionality in fixed tissue macrophages [6]. Many subsequent studies have shown that zymosans, particularly BG, increase the function of macrophages, neutrophils, basophils, mast cells, and other immunocytes [7]. Depending on the size and the origin, the molecular effects of BG are diverse. High molecular weight BGs appear to directly activate leukocytes and stimulate phagocytic, cytotoxic, and anti-microbial activities, leading to the production of many proinflammatory mediators, cytokines, and chemokines (IL-8, IL-1b, IL-6, and TNFα) [5]. Low molecular weight BGs are reported to induce the release of IL-8, IL-6, and nuclear transcription factors such as NF-kB and NF IL-6 [8]. BG stimulates and activates mast cells by binding with BG receptor on the surface of macrophages. Stimulation of 1,3-BG-receptors results in Ca2+ influx through receptor-operated channels. [910]. An increase in intracellular Ca2+ ([Ca2+]i) is necessary for BG-induced mast cell exocytosis, but whether this Ca2+ is derived from extracellular or intracellular pools is still controversial [11].
Mitochondria are key subcellular organelles which regulate the life and death of cells via producing ATP and triggering apoptosis signals. Beside, mitochondria regulate intracellular Ca2+ homeostasis by uptake and release of intracellular Ca2+ responded to various biological processes. Because mast cell activation and degranulation are highly ATP and Ca2+ dependent process, many studies demonstrated the important role of mitochondria in mast cell degranulation [121314151617]. However, those findings were mainly found in FcεRI-mediated degranulation. The mechanisms underlying BG-induced degranulation and the mitochondrial role in this process are not well known yet.
The present study aimed to investigate the role of cytosolic and mitochondrial calcium in BG-induced mast cell degranulation. Our results provide important evidence for the role of mitochondrial Ca2+ uniporter in the BG mediated by mast cell degranulation.
Eight-week-old C57BL mice were used for all experiments. Bone marrow-derived mast cells (BMBCs) were collected and cultured in Iscove's modified Dulbecco's medium (IMDM, Thermo Fisher, Waltham, USA ) supplemented with 15% fetal bovine serum (FBS, Thermo Fisher, Waltham, USA), 15 µM thioglycerol, 2 mM L-glutamine, stem cell factor (SCF; 50 ng/ml), and IL-3 (30 ng/ml); cells were incubated at 37℃, 95% O2, and 5% CO2. Media were changed every week. After 4 weeks, cells were used for experiments. All animal studies were approved by the Inje Medical University Animal Care and Use Committee.
Normal Tyrode's solution (NT) contained (in mM): 143.0 mM NaCl, 5.4 mM KCl, 1.8 mM CaCl2, 0.5 mM MgCl2, 5.5 mM glucose, and 5.0 mM HEPES (pH 7.4). Fluorescent probes were supplied by Molecular Probes (Eugene, Oregon, USA). The tryptase release kit was purchased from Chemicon (CA, USA). FITC anti-mouse FcεRI, PE anti-mouse CD117, and isotype controls were supplied by eBioscience (CA, USA). All other reagents were obtained from Sigma (St. Louis, MO, USA).
BMBCs were cultured as described above and collected every week to check the development of the mast cell population. Mast cells were confirmed by toluidine blue staining, flow cytometry analysis, and laser scanning confocal microscopy. For toluidine blue staining, cells were stained with toluidine blue for 10 min at room temperature, and then visualized under a light microscope connected to a camera. For flow cytometry analysis, cells were stained with phycoerythrin (PE) anti-mouse FcεRIa and fluo-rescein isothiocyanate (FITC) anti-mouse CD117 (c-Kit). Cells were then subjected to flow cytometry using the CellQuestPro with FL1 filter for FITC anti-mouse CD117 and FL2 filter for PE anti-mouse FcεRI [18]. For laser scanning confocal microscopy, cells were stained with FITC anti-mouse CD117, PE anti-mouse FcεRI, or both probes. Cells were then visualized using laser scanning confocal microscopy with excitation/emission filters for visualizing FITC anti-mouse CD117 (488/505 nm) and PE antimouse FcεRI (514/560 nm).
BMMC degranulation was stimulated with different types of stimulators including beta-1,3-glucan (BG, 0.1 mg/ml), peptidoglycan from Staphylococcus aureus (PGN, 100 µg/ml), or calcium ionophore A23187 (10 µM) in normal Tyrode's solution to compare the pathway specific degranulation responses in mast cell. BG, PGN or A23187 stimulates mast cell through BG-receptors [19], TLR-2 pathway [20] or direct increase of intracellular Ca2+ [21], respectively. To test the role of the mitochondrial calcium uniporter (MCU), we used ruthenium red (RuR, 5 µM) as a selective MCU inhibitor. Calcium-free solution was prepared by the addition of 1 mM EGTA.
Tryptase is a tetrameric serine proteinase that has emerged as a major component of mast cell granules. Tryptase was measured using a kit according to the manufacturer's instructions. Briefly, BMMCs were suspended in Tyrode's solution and stimulated as described above. After the desired period of activation, the samples were centrifuged at 800 × g to separate supernatant and pellet. The pellet was then sonicated and centrifuged to collect lysate. Both supernatant and lysate were used to evaluate tryptase concentration. Tryptase concentration was determined spectrophotometrically (EL-800, BioTek Instruments) at 405 nm. Mast cell degranulation was calculated as percentage of released tryptase relative to the total tryptase using the following equation:
Degranulation = Tryptasesup×100 / (Tryptasesup+Tryptaselysate).
To evaluate [Ca2+]i , BMMCs were stained for 30 min with 5 µM Fluo4-AM, a specific cell-permeant probe that binds to cytosolic Ca2+. To measure mitochondrial Ca2+, BMMCs were incubated with 5 µM Rhod2-AM, a selective indicator for mitochondrial Ca2+, for 1 hour at 4℃, and then briefly incubated for 5 min at room temperature.
Mitochondrial membrane potential (ΔΨm) was measured using tetramethylrhodamine ethyl ester perchlorate (TMRE). BMMCs were incubated with 2 µM TMRE for 15 min at room temperature. After staining, BMMCs were washed twice with NT buffer, and fluorescence was imaged using a laser scanning confocal microscope.
To Monitor Vesicle Degranulation, Bmmcs Were Stained With Lysotracker Red Dnd99 (Ltr) [22]. We Measured The Reduction In Total Cellular Fluorescence Intensity And Monitored Vesicle Movement Out Of The Cell Membrane. We Confirmed The Decline Of Ltr During Degranulation By Comparing With Tryptase Release.
BMMCs stained with fluorescent probes were placed on a poly-L-lysine-coated cover glass that was placed inside a perfusion chamber, and subjected to experimental protocols. Fluorescence was acquired using a confocal laser scanning microscope (inverted Axiovert 200M, Zeiss, Jena, Germany) at 200× and 400× magnification. Data were analyzed with LSM5 10META software (Zeiss, Jena, Germany). Fluo4-AM, Rhod2-AM, and TMRE were visualized with suitable filter sets at excitation/emission wavelengths at 488 nm/520 nm, 550 nm/580 nm, 514/560 nm, respectively.
Bone marrow-derived cells cultured for 4 weeks showed a high percentage of mast cells. Toluidine blue staining confirmed that more than 98±1% of the total population were BMMCs (Fig. 1A, B). Flow cytometry analysis indicated that 97±0.6% and 98.3±0.4% of cells were bound with anti-FcεRI and anti-c-Kit probes, respectively (Fig. 1C). Images obtained from laser scanning confocal microscopy also indicated that the samples contained a high percentage of mast cells, with more than 98% cells co-localizing FcεRI and c-Kit (Fig. 1D).
BG induced increases in both cytosolic and mitochondrial calcium ([Ca2+]c and [Ca2+]m, respectively) (Fig. 2A). After 30 min of BG treatment, [Ca2+]m increased to 293±61.5% and then declined to 195.5±22.9% after washout (50 min). The [Ca2+]i values for the same intervals were 147.1±11.2% and 134.5±8.9%, respectively. Analysis of TMRE-associated mitochondrial membrane potential (ΔΨm) showed that BG treatment increased TMRE intensity to 155.7±9.7% (at 30 min), which then declined to 58.4±11.1% (at 50 min washout) (Fig. 2B~E). PGN also significantly increased [Ca2+]m to 159.9±12.8% of that in control cells (Fig. 2F).
BMMC degranulation was evaluated by both tryptase release measurement and LysoTracker Red DND99 (LTR) probe methods. We visualized cells with confocal microscopy to observe vesicle release after incubating BMMC with BG (Fig. 3A). We observed two phases of exocytosis within the time frame of our experiments. The total LTR levels in mast cells decreased after a few minutes (<20 min), and again declined after 30 min since the start of BG treatment (Fig. 3B, C). We selected narrow areas at the cell periphery near the cell membrane to detect vesicle movement. Two phases of degranulation was illustrated in left panel of Fig. 3D. Fluorescence images showed that LTR-stained vesicles in the cytoplasm were still present after the BG-induced early phase of exocytosis of membrane proximal vesicles (Fig. 3D right).
BG treatment of BMMCs in the absence of extracellular Ca2+ (by adding 1 mM EGTA to the medium) resulted in less reduction of LTR fluorescence compared with that in normal Tyrode's medium (1.8 mM CaCl2) (Fig. 3E). The second degranulation phase (after 30 min) was blocked by the presence of 5 µM RuR (Fig. 3B and E).
The addition of 5 µM RuR inhibited tryptase release induced by A23187, PGC, and BG in the presence of normal extracellular Ca2+. RuR had a weaker effect on A23187-induced degranulation than on PGN- and BG-induced degranulation. The percentage of degranulation induced by A23187, PGN, and BG were 70.6, 59.5, and 64.3%, respectively, in the absence of RuR and 51.5, 35.5, and 29.3%, respectively, in the presence of RuR (Fig. 4). A23187-, PGN-, and BG-induced degranulation was significantly suppressed in the absence of extracellular Ca2+ (Fig. 4).
This study demonstrated that the mitochondrial calcium uniporter is an important regulator of Ca2+-dependent BG-induced mast cell degranulation. BGs are natural polysaccharides which are contained in the cell wall of yeast, fungi and seaweed. Various BGs can be classified according to molecular characteristics such as molecular mass, solubility, viscosity, and three-dimensional configuration. BG is widely used in various clinical applications including in DNA damage protection [23], antioxidative therapy [24], anti-cancer [25] and immune stimulation [19]. Among these clinical applications, the role of BG in mast cell stimulation and degranulation has been extensively studied due to its importance in cytokine-mediated immune response [2627282930]. Several mechanisms and components involved in mast cell degranulation have been identified, including the molecular receptor, signaling pathways, localization modulator, candidate ligands, and Ca2+ regulation [41031323334]. Cellular recognition of BG is suggested by the characterization of four types of BG receptors: scavenger receptor, CR3, lactosyl ceramide, and dectin-1 [19]. A recent study reported that dectin-1 was almost exclusively responsible for the BG-dependent nonopsonic recognition of zymosan by primary macrophages [34].
Intracellular and/or extracellular Ca2+ pools are determining factors of mast cell degranulation [121521]. During BG-mediated activation of a macrophage cell line, stimulation of 1,3-BG receptors via zymosan enhanced Ca2+ influx through PKC delta and receptor-operated Ca2+ channels without an increase in IP3 production [910]. Consistent with the findings of a previous study regarding macrophages, our results showed that BG treatment gradually increased [Ca2+]i in BMMCs (Fig. 2B and D).
Mitochondria are important regulators of mast cell functions including energy production [35], apoptosis [363738], Ca2+ mobility [39], and degranulation [404142]. Cross talk between mitochondria and cytosolic Ca2+ regulation has been suggested as an important mechanism regulating degranulation [121314151617]. Suzuki et al. suggested that the mitochondrial permeability transition pore (mPTP) is the fast-releasing Ca2+ channel [13] and MCU as a Ca2+ uptake channel. At low Ca2+ concentrations (0.1 µM), IP3-sensitive storage primarily uptake Ca2+, but at higher Ca2+ concentrations (>1 µM), Ca2+ uptake is primarily into mitochondria via MCU [43]. Inhibition of mitochondrial metabolism [1339] or targeted knockdown of MCU [17] significantly reduced FcεRI-mediated mast cell degranulation. Mitochondrial membrane potential is an important factor regulating FcεRI-mediated mast cell degranulation [13]. Under normal extracellular Ca2+ levels, a mitochondrial inhibitor and a mitochondrial uncoupler inhibit IgE-induced degranulation. In contrast, antimycin A and FCCP enhance degranulation in the absence of extracellular Ca2+. IgE-mediated stimulation did not induce ΔΨm depolarization during degranulation [13].
There was limited evidence for the role of mitochondria in BG-dependent mast cell activation. We demonstrated that BG increased both cytosolic and mitochondrial Ca2+ levels (Fig. 2). In addition, we observed that BG rapidly induced an approximately two-fold increase in ΔΨm (hyperpolarization), before gradually declining (depolarization). These results suggest that mitochondrial ΔΨm and [Ca2+]m are involved in BG-induced BMMC degranulation.
To understand the functional role of mitochondrial Ca2+ during BMMC degranulation, we used RuR to block MCU activity and observed an alteration in degranulation rate. BG treatment induces vesicle release in two phases. The first degranulation phase occurred within seconds after treatment and continued until 20 min after the start of degranulation. During this period, approximately 20~30% of all vesicles were released. The second degranulation phase occurred within 20~40 min after the start of degranulation, when approximately 70% of all vesicles were released. Both degranulation phases were inhibited in the absence of extracellular Ca2+, whereas pharmacological inhibition of MCU only attenuated the second degranulation phase (Fig. 3). Our result is in agreement with an earlier study by Dvorak et al., which reported that anaphylactic degranulation of guinea pig basophilic leukocytes progressed through two phases [44].
The tryptase release assay successfully detected that MCU inhibition suppressed BMMC degranulation can be induced by three different stimuli: (1) Ca2+ elevation induced by the Ca2+ ionophore A23187, (2) BG-mediated BG receptor activation, and (3) Toll-like receptor 2 (TLR2, PGN)-mediated stimulation. PGN is a TLR2-dependent mast cell stimulator that increases [Ca2+]i and induces release of TNF-α and ILs [20]. Although, BG and PGN have different target receptors in the plasma membrane, both BG and PGN increased mitochondrial Ca2+ (Fig. 2F) and tryptase release in a similar pattern (Fig. 4). Furthermore, removal of extracellular Ca2+ commonly attenuated the tryptase release in both treatment (Fig. 4), suggesting that intracellular Ca2+ level and MCU activity are major factors in elevating mitochondrial Ca2+. A similar BG and PGN mechanism is also responsible in regulating mitochondrial Ca2+ by enhancing [Ca2+]i.
RuR suppressed degranulation in BG- and PGN-treated cells to levels similar to Ca2+-depleted conditions, but the inhibitory effect was weaker in the presence of A23187. A23187 directly elevates the [Ca2+]i level to the extracellular level (1.8 mM), which is sufficient to induce continuous degranulation. Although we observed that MCU inhibition restricted the second degranulation phase of BMMCs, the underlying mechanism has yet to be elucidated.
Recent studies suggested a novel role of mitochondria in immunologic response, in which mitochondrial translocation to exocytosis sites is required for human mast cell degranulation and preformed TNF secretion [45], and wherein mitochondrial components are secreted from mast cell during degranulation inducing inflammatory response [46]. Ca2+ dependent mitochondrial transportation has been actively studied in the neuronal cells. It was demonstrated that mitochondrial matrix Ca2+ is an important signal that actively regulates mitochondrial transportation. And MCU or MCU-related miro1 proteins are potential regulators of mitochondrial translocation [474849]. Based on these studies, we suggest that MCU inhibition might block mitochondrial translocation to exocytosis sites, which inhibits the 2nd phase of degranulation. However, this hypothesis should be further confirmed by extensive experimental evidences.
In a clinical setting, the optimal regulation of immune response is crucial for patients afflicted with immunological diseases. Although BGs are relatively safe since these are considered as natural immunomodulators which do not overstimulate the immune system [50], the identification of regulatory mechanism or component is still very important for the fine tuning of mast cell immune systems.
In conclusion, the present study identifies the crucial role of MCU during Ca2+-dependent mast cell degranulation. This result suggests that the mitochondria are essential regulators of mast cell activation and immunomodulation. This knowledge will be relevant for the optimization of BG- induced clinical immune modulation.
ACKNOWLEDGMENTS
This study was supported by a grant from the Priority Research Centers Program and Basic Science Research Program through the National Research Foundation of Korea (NRF), Funded by the Ministry of Education, Science and Technology (2010-0020224, 2012R1A2A1A03007595 and NRF-2015R1D1A1A01057937), Republic of Korea.
Notes
References
2. Wedemeyer J, Galli SJ. Mast cells and basophils in acquired immunity. Br Med Bull. 2000; 56:936–955. PMID: 11359630.


3. Kulka M, Sheen CH, Tancowny BP, Grammer LC, Schleimer RP. Neuropeptides activate human mast cell degranulation and chemokine production. Immunology. 2008; 123:398–410. PMID: 17922833.


4. Roth K, Chen WM, Lin TJ. Positive and negative regulatory mechanisms in high-affinity IgE receptor-mediated mast cell activation. Arch Immunol Ther Exp (Warsz). 2008; 56:385–399. PMID: 19082920.


5. Williams D, Mueller A, Browder W. Glucan-based macrophage stimulators. Clin Immunother. 1996; 5:392–399.


6. Benacerraf B, Thorbecke GJ, Jacoby D. Effect of zymosan on endotoxin toxicity in mice. Proc Soc Exp Biol Med. 1959; 100:796–799. PMID: 13645722.


7. Williams JD, Topley N, Alobaidi HM, Harber MJ. Activation of human polymorphonuclear leucocytes by particulate zymosan is related to both its major carbohydrate components: glucan and mannan. Immunology. 1986; 58:117–124. PMID: 3710519.
8. Adams DS, Pero SC, Petro JB, Nathans R, Mackin WM, Wakshull E. PGG-Glucan activates NF-kappaB-like and NF-IL-6-like transcription factor complexes in a murine monocytic cell line. J Leukoc Biol. 1997; 62:865–873. PMID: 9400829.
9. Zhang GH, Helmke RJ, Mörk AC, Martinez JR. Regulation of cytosolic free Ca2+ in cultured rat alveolar macrophages (NR8383). J Leukoc Biol. 1997; 62:341–348. PMID: 9307072.
10. Mörk AC, Sun X, Liu X, Rodriguez D, Martinez JR, Castro R, Zhang GH. Regulation of (1-3)-beta-glucan-stimulated Ca2+ influx by protein kinase C in NR8383 alveolar macrophages. J Cell Biochem. 2000; 78:131–140. PMID: 10797572.
11. Sengeløv H, Kjeldsen L, Borregaard N. Control of exocytosis in early neutrophil activation. J Immunol. 1993; 150:1535–1543. PMID: 8381838.
12. Mohr FC, Fewtrell C. The effect of mitochondrial inhibitors on calcium homeostasis in tumor mast cells. Am J Physiol. 1990; 258:C217–C226. PMID: 2137675.


13. Suzuki Y, Yoshimaru T, Inoue T, Ra C. Mitochondrial Ca2+ flux is a critical determinant of the Ca2+ dependence of mast cell degranulation. J Leukoc Biol. 2006; 79:508–518. PMID: 16365155.
14. Suzuki Y, Yoshimaru T, Inoue T, Nunomura S, Ra C. The high-affinity immunoglobulin E receptor (FcepsilonRI) regulates mitochondrial calcium uptake and a dihydropyridine receptor-mediated calcium influx in mast cells: Role of the FcepsilonRIbeta chain immunoreceptor tyrosine-based activation motif. Biochem Pharmacol. 2008; 75:1492–1503. PMID: 18243160.
15. Ma HT, Beaven MA. Regulation of Ca2+ signaling with particular focus on mast cells. Crit Rev Immunol. 2009; 29:155–186. PMID: 19496745.
16. Inoue T, Suzuki Y, Ra C. Epigallocatechin-3-gallate inhibits mast cell degranulation, leukotriene C4 secretion, and calcium influx via mitochondrial calcium dysfunction. Free Radic Biol Med. 2010; 49:632–640. PMID: 20510351.


17. Furuno T, Shinkai N, Inoh Y, Nakanishi M. Impaired expression of the mitochondrial calcium uniporter suppresses mast cell degranulation. Mol Cell Biochem. 2015; 410:215–221. PMID: 26350567.


18. Gurish MF, Friend DS, Webster M, Ghildyal N, Nicodemus CF, Stevens RL. Mouse mast cells that possess segmented/multi-lobular nuclei. Blood. 1997; 90:382–390. PMID: 9207474.


19. Akramiene D, Kondrotas A, Didziapetriene J, Kevelaitis E. Effects of beta-glucans on the immune system. Medicina (Kaunas). 2007; 43:597–606. PMID: 17895634.
20. Supajatura V, Ushio H, Nakao A, Akira S, Okumura K, Ra C, Ogawa H. Differential responses of mast cell Toll-like receptors 2 and 4 in allergy and innate immunity. J Clin Invest. 2002; 109:1351–1359. PMID: 12021251.
21. Hosokawa J, Suzuki K, Nakagomi D, Tamachi T, Takatori H, Suto A, Nakajima H. Role of calcium ionophore A23187-induced activation of IkappaB kinase 2 in mast cells. Int Arch Allergy Immunol. 2013; 161(Suppl 2):37–43.


22. Mizuno K, Tolmachova T, Ushakov DS, Romao M, Abrink M, Ferenczi MA, Raposo G, Seabra MC. Rab27b regulates mast cell granule dynamics and secretion. Traffic. 2007; 8:883–892. PMID: 17587407.


23. Slamenová D, Lábaj J, Krizková L, Kogan G, Sandula J, Bresgen N, Eckl P. Protective effects of fungal (1-->3)-beta-D-glucan derivatives against oxidative DNA lesions in V79 hamster lung cells. Cancer Lett. 2003; 198:153–160. PMID: 12957353.
24. Krizková L, Duracková Z, Sandula J, Slamenová D, Sasinková V, Sivonová M, Krajcovic J. Fungal beta-(1-3)-D-glucan derivatives exhibit high antioxidative and antimutagenic activity in vitro. Anticancer Res. 2003; 23:2751–2756. PMID: 12894570.
25. Nasrollahi Z, Mohammadi SR, Mollarazi E, Yadegari MH, Hassan ZM, Talaei F, Dinarvand R, Akbari H, Atyabi F. Functionalized nanoscale β-1,3-glucan to improve Her2+ breast cancer therapy: In vitro and in vivo study. J Control Release. 2015; 202:49–56. PMID: 25597638.
26. Zanoni I, Granucci F. Regulation and dysregulation of innate immunity by NFAT signaling downstream of pattern recognition receptors (PRRs). Eur J Immunol. 2012; 42:1924–1931. PMID: 22706795.


27. Sakurai A, Yamaguchi N, Sonoyama K. Cell Wall Polysaccharides of Candida albicans Induce Mast Cell Degranulation in the Gut. Biosci Microbiota Food Health. 2012; 31:67–70. PMID: 24936351.
28. Kimura Y, Chihara K, Honjoh C, Takeuchi K, Yamauchi S, Yoshiki H, Fujieda S, Sada K. Dectin-1-mediated signaling leads to characteristic gene expressions and cytokine secretion via spleen tyrosine kinase (Syk) in rat mast cells. J Biol Chem. 2014; 289:31565–31575. PMID: 25246527.


29. Cleary JA, Kelly GE, Husband AJ. The effect of molecular weight and beta-1,6-linkages on priming of macrophage function in mice by (1,3)-beta-D-glucan. Immunol Cell Biol. 1999; 77:395–403. PMID: 10540205.
30. Kolaczkowska E, Seljelid R, Plytycz B. Role of mast cells in zymosan-induced peritoneal inflammation in Balb/c and mast cell-deficient WBB6F1 mice. J Leukoc Biol. 2001; 69:33–42. PMID: 11200065.
31. Marshall JS, Stead RH, McSharry C, Nielsen L, Bienenstock J. The role of mast cell degranulation products in mast cell hyperplasia .I. Mechanism of action of nerve growth factor. J Immunol. 1990; 144:1886–1892. PMID: 2106555.
32. Dvorak AM, Galli SJ, Schulman ES, Lichtenstein LM, Dvorak HF. Basophil and mast cell degranulation: ultrastructural analysis of mechanisms of mediator release. Fed Proc. 1983; 42:2510–2515. PMID: 6840300.
33. Nishida K, Yamasaki S, Ito Y, Kabu K, Hattori K, Tezuka T, Nishizumi H, Kitamura D, Goitsuka R, Geha RS, Yamamoto T, Yagi T, Hirano T. Fc{epsilon}RI-mediated mast cell degranulation requires calcium-independent microtubule-dependent translocation of granules to the plasma membrane. J Cell Biol. 2005; 170:115–126. PMID: 15998803.
34. Brown GD, Taylor PR, Reid DM, Willment JA, Williams DL, Martinez-Pomares L, Wong SY, Gordon S. Dectin-1 is a major beta-glucan receptor on macrophages. J Exp Med. 2002; 196:407–412. PMID: 12163569.
35. Weatherly LM, Shim J, Hashmi HN, Kennedy RH, Hess ST, Gosse JA. Antimicrobial agent triclosan is a proton ionophore uncoupler of mitochondria in living rat and human mast cells and in primary human keratinocytes. J Appl Toxicol. 2015; DOI: 10.1002/jat.3209. [Epub ahead of print].


36. Yoshimaru T, Suzuki Y, Inoue T, Nishida S, Ra C. Extracellular superoxide released from mitochondria mediates mast cell death by advanced glycation end products. Biochim Biophys Acta. 2008; 1783:2332–2343. PMID: 18822320.


37. Inoue T, Suzuki Y, Mizuno K, Nakata K, Yoshimaru T, Ra C. SHP-1 exhibits a pro-apoptotic function in antigen-stimulated mast cells: positive regulation of mitochondrial death pathways and negative regulation of survival signaling pathways. Mol Immunol. 2009; 47:222–232. PMID: 19875169.


38. Suzuki Y, Yoshimaru T, Inoue T, Ra C. Ca v 1.2 L-type Ca2+ channel protects mast cells against activation-induced cell death by preventing mitochondrial integrity disruption. Mol Immunol. 2009; 46:2370–2380. PMID: 19447492.
39. Takekawa M, Furuno T, Hirashima N, Nakanishi M. Mitochondria take up Ca2+ in two steps dependently on store-operated Ca2+ entry in mast cells. Biol Pharm Bull. 2012; 35:1354–1360. PMID: 22863937.


40. Erlich TH, Yagil Z, Kay G, Peretz A, Migalovich-Sheikhet H, Tshori S, Nechushtan H, Levi-Schaffer F, Saada A, Razin E. Mitochondrial STAT3 plays a major role in IgE-antigen-mediated mast cell exocytosis. J Allergy Clin Immunol. 2014; 134:460–469. PMID: 24582310.


41. Zhang B, Weng Z, Sismanopoulos N, Asadi S, Therianou A, Alysandratos KD, Angelidou A, Shirihai O, Theoharides TC. Mitochondria distinguish granule-stored from de novo synthesized tumor necrosis factor secretion in human mast cells. Int Arch Allergy Immunol. 2012; 159:23–32. PMID: 22555146.


42. Sadroddiny E, Ai J, Carroll K, Pham TK, Wright P, Pathak A, Helm B. Protein profiling of the secretome of FcεRI activated RBL-2H3.1 cells. Iran J Immunol. 2012; 9:1–31. PMID: 22426164.
43. Ali H, Maeyama K, Sagi-Eisenberg R, Beaven MA. Antigen and thapsigargin promote influx of Ca2+ in rat basophilic RBL-2H3 cells by ostensibly similar mechanisms that allow filling of inositol 1,4,5-trisphosphate-sensitive and mitochondrial Ca2+ stores. Biochem J. 1994; 304:431–440. PMID: 7998977.
44. Dvorak AM, Galli SJ, Morgan E, Galli AS, Hammond ME, Dvorak HF. Anaphylactic degranulation of guinea pig basophilic leukocytes. I. Fusion of granule membranes and cytoplasmic vesicles formation and resolution of degranulation sacs. Lab Invest. 1981; 44:174–191. PMID: 6162057.
45. Zhang B, Alysandratos KD, Angelidou A, Asadi S, Sismanopoulos N, Delivanis DA, Weng Z, Miniati A, Vasiadi M, Katsarou-Katsari A, Miao B, Leeman SE, Kalogeromitros D, Theoharides TC. Human mast cell degranulation and preformed TNF secretion require mitochondrial translocation to exocytosis sites: relevance to atopic dermatitis. J Allergy Clin Immunol. 2011; 127:1522–1531.e8. PMID: 21453958.


46. Zhang B, Asadi S, Weng Z, Sismanopoulos N, Theoharides TC. Stimulated human mast cells secrete mitochondrial components that have autocrine and paracrine inflammatory actions. PLoS One. 2012; 7:e49767. PMID: 23284625.


47. Wang X, Schwarz TL. The mechanism of Ca2+-dependent regulation of kinesin-mediated mitochondrial motility. Cell. 2009; 136:163–174. PMID: 19135897.
48. Chang KT, Niescier RF, Min KT. Mitochondrial matrix Ca2+ as an intrinsic signal regulating mitochondrial motility in axons. Proc Natl Acad Sci U S A. 2011; 108:15456–15461. PMID: 21876166.
49. Niescier RF, Chang KT, Min KT. Miro, MCU, and calcium: bridging our understanding of mitochondrial movement in axons. Front Cell Neurosci. 2013; 7:148. PMID: 24058334.


Fig. 1
Characterization of mouse bone marrow-derived mast cells (BMMCs) cultured for 4 weeks.
(A) Microscopic image of BMMCs (×400 magnification). (B) Toluidine blue-stained image of BMMCs (×400 magnification). (C) Flow cytometry analysis of Fcε RI and c-Kit-positive populations in BMMCs. (D) Confocal microscopy images of cells with positive staining for c-Kit (green) and Fcε RI (red) (×200 magnification).
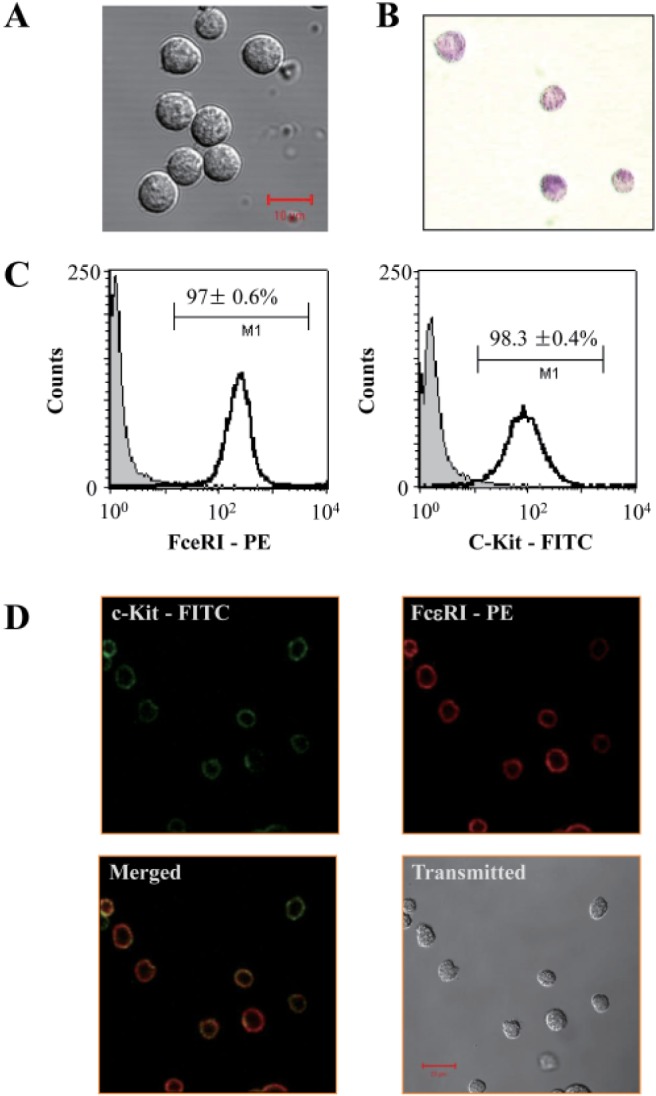
Fig. 2
Beta-1,3-glucan stimulation increases cytosolic and mitochondrial Ca2+ levels in BMMCs.
(A) Confocal microscopy images of BMMCs (green, 5 µM Fluo4-AM; red, 5 µM Rhod2-AM). (B) Fluorescence intensity trace of mitochondrial Ca2+, cytosolic Ca2+, and mitochondrial membrane potential (ΔΨm) during BG stimulation of mast cell degranulation. (C) Normalized changes in mitochondrial Ca2+ levels monitored in BMMCs stained with Rhod2-AM. (D) Normalized changes in cytosolic Ca2+ levels monitored in BMMCs stained with Fluo4-AM. (E) Normalized changes in ΔΨm monitored in TMRE-stained BMMCs. a, b, and c represent 5, 30, and 50 min, respectively. (F) Normalized comparison of mitochondrial Ca2+ ele vation induced by BG or PGN treatment. (C~E) *p<0.05 vs. time point a, †p<0.05 vs. time point b. (F) *p<0.05 vs. Control

Fig. 3
BG-induced mast cell degra nulation was inhibited by ruthenium red (RuR), a mitochondrial calcium uniporter (MCU) blocker.
(A) Repre sentative fluorescence images of BMMCs stained with LysoTracker Red (LTR). BG treatment gradually reduced LTR intensity. (B) Normalized changes in LTR fluorescence mediated by BG or BG+RuR treatment. (C) Two-dimensional fluorescence intensity image (a, b, and c represent 5, 30, and 50 min, res pectively). (D) Left: Illustration of two phases of degranulation. ① membrane proximal vesicle degranulation (<20 min), ② cytoplasmic vesicle to be tran slocated to membrane for degranulation (>20 min). Right: Cytoplasmic LTR- stained vesicle after BG-induced early phase degranulation of membrane proximal vesicles (>20 min). (E) Changes in the time course of LTR intensity during mast cell degranulation.
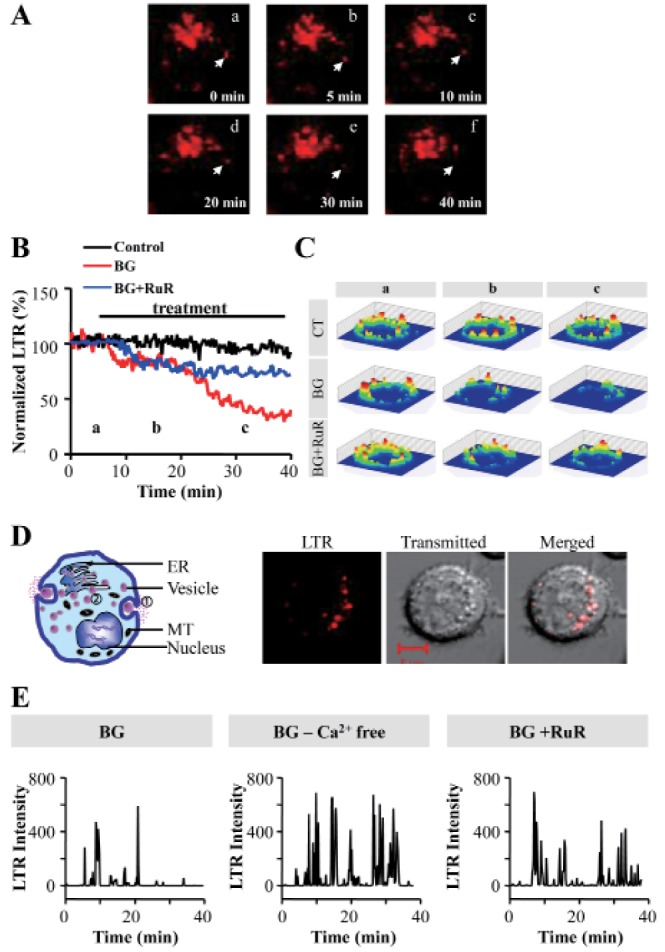