Abstract
Zinc has been considered as a vital constituent of proteins, including enzymes. Mobile reactive zinc (Zn2+) is the key form of zinc involved in signal transductions, which are mainly driven by its binding to proteins or the release of zinc from proteins, possibly via a redox switch. There has been growing evidence of zinc's critical role in cell signaling, due to its flexible coordination geometry and rapid shifts in protein conformation to perform biological reactions. The importance and complexity of Zn2+ activity has been presumed to parallel the degree of calcium's participation in cellular processes. Whole body and cellular Zn2+ levels are largely regulated by metallothioneins (MTs), Zn2+ importers (ZIPs), and Zn2+ transporters (ZnTs). Numerous proteins involved in signaling pathways, mitochondrial metabolism, and ion channels that play a pivotal role in controlling cardiac contractility are common targets of Zn2+. However, these regulatory actions of Zn2+ are not limited to the function of the heart, but also extend to numerous other organ systems, such as the central nervous system, immune system, cardiovascular tissue, and secretory glands, such as the pancreas, prostate, and mammary glands. In this review, the regulation of cellular Zn2+ levels, Zn2+-mediated signal transduction, impacts of Zn2+ on ion channels and mitochondrial metabolism, and finally, the implications of Zn2+ in health and disease development were outlined to help widen the current understanding of the versatile and complex roles of Zn2+.
Zinc is the 24th most abundant element in the Earth's crust, with five naturally occurring isotopes. In 1869, scientists first described zinc as an essential nutrient for the growth of living organisms, due to the lack of its storage in major organs [1]. Zinc deficiency had been identified as a causative factor in the delayed sexual development and stunted growth in humans, because of the vital role of zinc in both male and female hormone production [2]. Normally, the human body contains 2~3 g of zinc (approx. 15.5 µM), 0.1% of which is exchanged daily [3]. Cellular zinc is most abundant in the cytoplasm (50%), followed by the nucleus (30~40%), and occurs least in the membrane (10%) [4]. Metalloproteomic analysis has revealed that prokaryotes use approximately 83% of their zinc proteins to carry out enzymatic catalysis, whereas eukaryotes utilize practically equal ratios of zinc-related proteins in catalytic reactions (47%) and DNA transcription (44%), while the remaining are involved in transport (5%) and signaling (3%) [5]. According to Costello et al., zinc occurring in mammalian biological systems can be classified into at least three forms, depending on its molecular behavior [6]: first, the non-exchangeable and non-reactive immobile zinc, which is tightly bound predominantly to proteins and comprises about 54% of the zinc pool; second, the "mobile reactive zinc" that is loosely bound to a ligand and is an exchangeable reactive pool of zinc (44.7%); and third, the reactive pool of zinc or the free zinc (<1%, ~5 pM~1 nM) (Fig. 1). Moreover, five types of Zn2+ sites are apparent from the examination of Zn2+-containing proteins: structural, multinuclear (clustered), catalytic (over 300 various enzymes), transport, and interprotein [789]. This reinforces the possibility of varied activities of zinc in cellular processes. Here, Zn2+ represents an exchangeable reactive pool and a reactive pool of zinc (or free zinc). The availability of Zn2+ inside the cell influences protein function, more evidently via interprotein Zn2+ binding sites, which responds to Zn2+ in a concentration-dependent manner [9]. Signaling cascades are induced not by non-exchangeable zinc (tightly bound to proteins), but by mobile reactive or free zinc.
Zn2+ can act as an efficient Lewis acid and is often integrated to the amino acid side chains of aspartic acid, glutamic acid, cysteine, and histidine. Among them, cysteine has an important role in the cellular mobility of zinc in proteins. Thus, its flexible coordination geometry with proteins allows a rapid shift of conformations in order to perform certain biological reactions [10111213]. Zinc, while an essential requirement for good health, can be harmful when reaching excessive and toxic levels, or when its homeostasis is perturbed by the suppressed absorption of other metal ions, such as copper and iron [914]. The impact of Zn2+ concentrations on protein function in the cell is summarized in Fig. 1. The importance and complexity of Zn2+ activity has been presumed to parallel the degree of calcium's participation in cellular processes [815]. This study aims to summarize the versatile roles of Zn2+, especially on cellular signaling, to better understand its physiological and pathophysiological roles in the heart and other organ systems. In this review, we will briefly summarize: (1) the regulation of cellular Zn2+, (2) Zn2+-mediated cellular functions, (3) Zn2+ activity on ion channels, (4) Zn2+ and mitochondrial metabolism, and finally, (5) the implications of Zn2+ on disease and health.
Zinc homoeostasis is maintained through mechanisms that regulate the gene expressions involved in zinc homoeostatic pathways; Zn2+ transporters (Official name: Solute Carrier Family 30A, SLC30A, ZnTs), Zn2+ importers (Official name: Solute carrier Family 39A, SLC39A, ZIPs), and the intracellular zinc-binding protein, metallothionein (MT) [812161718]. An outline of cellular Zn2+ movement by MTs, ZnTs, and ZIPs is depicted in Fig. 2. Dietary zinc is typically absorbed in the intestine and MT synthesis in mucosal cells is triggered by both fasting and high luminal zinc concentrations [1920]. Generally, Zn2+ is impermeable across the plasma membrane. Hence, after the uptake of Zn2+ via ZIPs in the small intestine, the distribution of Zn2+ is carried out through the plasma membrane, where it is bound by proteins, mostly albumin (~77%), which is the major protein component of blood plasma and is responsible for the circulatory transport of a range of small molecules. The remaining Zn2+ is bound to globulin (~20%) [2122].
Intracellular Zn2+ must be tightly regulated to prevent the adverse effects of zinc deficiency or toxicity, but since it cannot pass through biological membranes unassisted, two families of zinc-transport proteins aid in this process. A total of 10 ZnT and 14 ZIP gene loci in humans allows for the fine-tuning of Zn2+ distribution among tissues and intracellular organelles, such as the endoplasmic reticulum (ER) or Golgi apparatus [1523242526]. ZnT and ZIP proteins are energy independent, and their production is regulated by Zn2+ levels [27]. The fundamental role of Zn2+ flux from intracellular stores can be anticipated from the ubiquity of ZIP7 among different organisms and cell types [28], since Zn2+ release from stores is stimulated without the addition of exogenous zinc [15]. Most of the ZIP family is predicted to have eight transmembrane domains with extracellular and intravesicular amino- and carboxyl-termini, while most ZnT transporters have six. The metal binding domain of ZnTs is represented by a common histidine-rich loop [29], and this transporter promotes zinc efflux from cells or into intracellular vesicles to reduce intracellular Zn2+ availability. However, ZIPs do the opposite by enabling the uptake of extracellular Zn2+, and possibly releasing vesicular Zn2+ into the cytoplasm. These transporter families exhibit tissue-specific expression and heterogeneous sensitivity to dietary zinc deficiency and excess, as well as to other physiologic stimuli via hormones and cytokines [30].
MT is a small protein (~14 kDa) and comprises a family of cysteine (cys)-rich proteins [31]. The human body synthesizes MT in large quantities, mainly in the liver, kidney, intestine, and pancreas. MTs are localized in the membrane of the Golgi apparatus. The thiol group of its cysteine residues (~30% of its amino acid residues) allows MTs to bind both physiological and xenobiotic heavy metals. The homeostasis of zinc in a system is achieved by the coordination of MT with metal-free thionein, whose production is induced by increased zinc availability. The specific knockout of MT genes in mice with multiple MT-1 genes does not exhibit major developmental defects, suggesting a minimal role in normal growth and development; however, during conditions of major infection and inflammation, MTs have been known to provide survival advantages [32].
MT gene expression is triggered by various stimuli, and the MT promoter region has specific sequences for metal response elements (MRE), glucocorticoid response elements, GC-rich boxes, basal level elements, and thyroid response elements [32]. Transcriptional activation also occurs at higher zinc levels, as observed in the gene regulatory response mediated by MRE-binding transcription factor 1 (MFT1) to copies of the MRE consensus sequence in the promoter region [33]. MT also serves as a free radical scavenger [31]. Mammalian MT-1/MT-2 isoforms are involved in zinc homeostasis and protection against heavy metal toxicity and oxidative stress. MT-3 is expressed mainly in neurons, but also in glia [34], while MT-4 is mostly present in differentiating stratified squamous epithelial cells [31].
As extracellular Zn2+ enters the cell, it is immediately buffered within a 'zinc muffler' via a still unidentified Zn2+ transporter, and is then translocated into an intracellular zinc store, such as the ER or Golgi [35]. The Zn2+ is then released into the cytosol from the ER through the action of ZIP7, creating a zinc wave [36]. Mitochondria are another possible site of intracellular zinc sequestration. Zn2+ can be released from zinc-bound MT, physiological Zn2+ ligands [37], cysteine, histidine, glutathione (GSH), aspartate, glutamate, and glycine, in an acidic condition such as in ischemia [3839], from ER and Golgi through ZnTs, mitochondria with unknown mechanisms [40], or acidic organelles [41]. The partial cationic character of Zn2+ enables it to attract anionic prooxidants, such as peroxynitrite (ONOO-). For example, zinc is released from endothelial nitric oxide synthase (eNOS) upon exposure to peroxynitrite, which results in dimer disruption and enzyme uncoupling [42]. Maret and colleagues demonstrated that the presence of GSH enabled a higher transfer rate, and caused the release of more of the available Zn2+ from MT by glutathione disulfide (GSSG), and, thereafter, can facilitate Zn2+ removal and addition to inhibitory sites on enzymes involved in glycolysis and signal transduction [43]. A pH-dependent release of Zn2+ by cytosolic proteins is evident with cysteine, wherein the liberation of Zn2+ rapidly accelerates when the pH is decreased from 6.6 to 6.1 [37]; however, MTs are not likely to release Zn2+ at the same pH trend [39] and Zn2+ release from GSH-Zinc is less sensitive to an acidic pH (pH 6.1) [37]. At physiological pH, cytosolic proteins and ligands prevent large increases in intracellular Zn2+ by rapidly buffering the Zn2+ released from the mitochondria. When the mitochondrial matrix pH drops from 8 to 7, GSH starts to release Zn2+, which then leaks into the cytosol. At a pH of 7, ligands, such as MTs and cysteine, intercept the released Zn2+; only after the pH drops below 6.6 and cysteine loses its affinity for Zn2+ does the elevation of intracellular Zn2+ levels become evident [37].
The role of Zn2+ in signal transduction pathways has recently become a subject of great interest, since numerous signaling pathways are influenced in the presence or loss of Zn2+ [12]. Binding of Zn2+ has been observed to prompt protein structural rearrangement, which eventually affects protein function [9]. Fukada et al. suggested that signaling evoked by intracellular Zn2+ may be classified by time windows, as transcription-independent early Zn2+ signaling happened within several minutes and transcription-dependent late Zn2+ signaling happened several hours after Zn2+ exposure, based on the actions of immune cells [12]. However, it remains unclear whether this Zn2+-mediated signaling is sequential from early to late Zn2+ signaling, or whether both early and late Zn2+ signaling are separate or combinatory.
In cardiomyocytes or other cells, the intracellular zinc concentrations are 100-fold less than those of intracellular Ca2+ [444]. The relatively low abundance of Zn2+ in cardiomyocytes suggests its availability as second messengers for gene expression [45]. The inhibition of cyclic AMP (cAMP) signaling by Zn2+ has been attributed to both an inhibition of adenylyl cyclase (AC), particularly the AC5 and AC6 isoforms [4647] that are present in the cardiomyocytes [48], and of the function of the Gs alpha subunit (Gαs). Zn2+ induces aggregates of pure tubulin, implying the activation of G proteins by tubulin dimers [49]. This possible regulation of cAMP signaling by Zn2+ will provide complex outcomes of cAMP and cAMP-protein kinase A signaling, which are initiated by various kinds of chemicals and hormones. Therefore, cAMP-mediated signaling may be influenced or amplified at different levels of cellular Zn2+.
Protein tyrosine phosphatase 1B (PTP1B) inhibits phosphoinositide 3-kinase, a key enzyme in the insulin and leptin signaling pathways, making it a negative regulator of both signaling pathways [50]. Zn2+ binds to the catalytic pocket of the phosphointermediate form of PTP1B at a nanomolar range [51], and works as an inhibitor of protein tyrosine phosphatases (PTPs) on account of an active site HC(X5)R signature motif and the nucleophilic attack of the phosphorus atom of the phosphate group by the sulfur ion of the cysteine thiol in the first step of the dephosphorylation reaction and/or formation of inactive dimers between thiolates of PTPs and zinc [1651]. ER-bound PTP1B is proposed to be inhibited by ZIP7-mediated Zn2+ release from the ER, because the co-localization of ZIP7 and PTP1B does not require a global increase in cytosolic Zn2+ in order to inhibit the enzyme. The inhibitory effect of Zn2+ on PTPs implicates zinc-induced signaling in the increase in tyrosine phosphorylation of insulin receptor (IR), insulin-like growth factor 1 receptor (IGF-1R), epidermal growth factor receptor (EGFR), or unrecognized receptor tyrosine kinases, depending on the cell type [52]. Moreover, both insulin and adiponectin directly work together with Zn2+, affecting their oligomerization, and hence their activities [21]. Therefore, an elevation in disturbances in intracellular Zn2+ status observed in metabolic disorders, such as obesity and diabetes, may directly impact leptin signaling by PTP1B inhibition [53]. In insulin-resistant rodent models, key regulators of the insulin signaling pathway, extracellular-signal-regulated kinases 1 and 2 (ERK1/2) and protein kinase B (PKB), were activated by Zn2+, and insulin-mimetic and anti-diabetic properties were also observed [52].
The cell's response to a perturbation in its surrounding environment heavily relies on the regulation of protein activity. Reactive disulfide bonds act as molecular switches, and proteins with closely spaced cysteines or cysteine-coordinating Zn2+ centers, including MT, may reveal that thiol-disulfide exchanges play a much more widespread role in protein regulation at the post-translational level than had been previously anticipated [54]. Intracellular Zn2+ levels can be increased by thiol-reactive oxidants. In cardiomyocytes, reactive oxygen species (ROS) are major contributors to a rapid increase in intracellular Zn2+ levels, due to the mobilization of Zn2+ from intracellular stores. Moreover, during oxidative stress, including ultraviolet A irradiation, Zn2+ is released from MTs, either by nitrosylation or thiol ligand oxidation [5556]. Intracellular Zn2+ may also contribute to oxidant-induced alterations of excitation-contraction coupling [55], changes in signal transduction, and alterations of mitochondrial function due to modification of protein structure or functions by Zn2+ release, or its binding to proteins [29]. These findings explain why antioxidant intervention, resulting in the prevention of both Zn2+ and Ca2+ leaks, is helpful against diabetic heart disease [4557]. Zn2+ plays a central role in the redox signaling network, in that when it binds to a sulfur ligand, it generates a redox-active environment, which allows conformational changes of the proteins it is attached to, activating several signaling molecules. For example, the activation of protein kinase C (PKC) could be initiated by the release of Zn2+ from its zinc finger regulatory domain by lipid second messengers or oxidation [58]. Another example is the Kv4.1 channel, a voltage-gated potassium channel that is a key regulator of membrane excitability in neuronal and cardiac tissues [5960]. Under physiological conditions, the native Kv4 channel complex activity is regulated by thiol-disulfide exchange reactions at the Zn2+ site upon exposure to reactive nitrosative or oxygen species. Intracellular nitric oxide (NO) rapidly inhibits the Kv4.1 channel in a Zn2+-dependent manner through a disulfide bridge across the T1-T1 interface (Cys110 to Cys132), which may be responsible for the nitrosative/oxidative modulation of rapidly inactivating (A-type) K+ currents in the heart and smooth muscle, as well as in neurons [59]. Other Kv2 and Kv3 channels share the similar T1-T1 Zn2+ site, and thus they also can be regulated by zinc-dependent redox modulation.
Numerous proteins are targets of Zn2+ binding, and this direct binding of Zn2+ to proteins is able to directly alter the function of proteins, including ion channels. Zn2+ is able to induce changes of the ionic current and significantly impede the activation kinetics of most K+ channels through its direct binding [60]. In addition, Zn2+ is among the most potent inhibitors of voltage-gated proton (H+) and calcium channels [6162]. The abundance of potential synaptic targets and the localization of Zn2+ in synaptic vesicles all seem to point to a role of Zn2+ in synaptic transmission [63]. The entry of Zn2+ in cortical neurons is mediated by L- and N-type voltage-gated Ca2+ channels, which may be enhanced by an acidic extracellular pH [64], whereas Zn2+ entry into heart cells is via dihydropyridine-sensitive Ca2+ channels and is reliant on electrical stimulation [65]. In the dorsal root ganglion (DRG) neurons, the rise in nanomolar concentrations of intracellular Zn2+ can activate transient receptor potential ankyrin 1 (TRPA1), which is a sensory receptor for environmental irritants and both oxidative and thiol-reactive compounds, possibly through interaction with the intracellular cysteine and histidine residues of TRPA1, and thus potentiates the perception of discomfort [6667].
Mitochondrial enzymes are also influenced by Zn2+ or oxidative stress-related Zn2+ release, and its dysregulation can take a toll on mitochondrial metabolism [40686970]. Although there is limited information available regarding Zn2+-dependent mitochondrial functions, changes in mitochondrial metabolism could be introduced by the direct binding of Zn2+ to mitochondrial proteins or the release of Zn2+ from its bound proteins, due to oxidative stress or redox switches, which result in an alteration of its enzymatic activity and function [71]. In the mitochondrial matrix, GSH has a more prominent action in Zn2+ chelation than it does in the cytosol. The application of a sulfhydryl reactive agent, N-ethylmaleimide, leads to a major intracellular Zn2+ release, indicating a large amount of zinc storage in Zn2+-cysteine complexes [3972]. While GTP binds to MT [73], ATP also binds to mammalian MT-2 [74]. Thus, it has been proposed that released Zn2+ from MT may be involved in the inhibition of ADP-stimulated respiration, or the formation of an ATP-Zn2+ complex, which is a preferred cofactor of pyridoxal kinase and flavokinase, which will be able to influence energy metabolism [74].
Zinc can inhibit mitochondrial electron transport by binding Zn2+ in complexes [7576] and stimulating a burst of mitochondrial ROS production [77]. Several zinc-regulated enzymes have been identified. Fructose 1, 6-diphosphatase, glyceraldehyde 3-phosphate dehydrogenase, aldehyde dehydrogenase, and lipoamide dehydrogenase (LADH) are inhibited by nanomolar concentrations of Zn2+ [7178]. Inside the mitochondrial matrix, an elevated Zn2+ concentration interrupts the Krebs cycle and mitochondrial energy metabolism by inhibiting the production of a reduced form of nicotinamide adenine dinucleotide (NADH) production. Besides, the Zn2+ pool in prostate mitochondria (0.1~1 µg zinc/mg protein) is essential for inhibiting mitochondrial aconitase in the Krebs cycle, possibly through MT-mediated Zn2+ transfer [79], which results in the accumulation of citrate for secretion into prostatic fluid [8081]. In neurons, excessive Zn2+ could induce mitochondrial swelling and ROS release. However, whether or not this induced swelling also occurs in the liver remains controversial [8283].
In mitochondria-mediated apoptosis, Zn2+ increases the Bax-associated mitochondrial pore forming process that releases cytochrome C, activating the caspase cascade that leads to apoptosis [84]. Zn2+ also increases the gene expression and cellular production of Bax and the Bax/Bcl-2 ratio, which facilitates the mitochondrial Bax-associated apoptogenic effect [85]. Zn2+ chelation at the onset of neuronal ischemia attenuates mitochondrial cytochrome C release and downstream caspase-3 activation [86]. In contrast to brain ischemia [38], Zn2+ is considered as an important modulator involved in cardioprotection upon reperfusion, since several suggested cardioprotectants share zinc activation [10].
In conjunction with the versatile roles of Zn2+, the differential expression of MT, ZnT, or ZIP participates in various kinds of pathological states, including inflammation [1232], neurodegenerative diseases, cancer, myocardial hypertrophy, and environmental metal exposure, since intracellular Zn2+ levels are largely influenced by these Zn2+ regulatory proteins [31]. The brief representation of Zn2+ regulation and possible outcomes is summarized in Fig. 3. Generally, dysregulated zinc metabolism in tissues with an intricate Zn2+ requirement is associated with metabolic diseases, such as cancer, diabetes, and infertility [9121487888990]. Aside from inadequate dietary zinc intake, zinc deficiency may result from impaired absorption, resorption, or increased excretion of Zn2+. Conditions such as chronic diarrhea, widespread burns, or wounds also accelerate endogenous zinc losses [91]. Alcoholics also frequently develop zinc deficiency, since alcohol causes the body to lose critical Zn2+ stores through the kidneys.
In the mammalian brain, vesicular zinc is co-localized with glutamate in a subset of glutamatergic nerve terminals throughout the forebrain. Zn2+ also shows anti-depression effects [92], which are associated with the action of G protein-coupled receptor 39 (GPR39), which is recognized as a Zn2+ receptor, and is a potent stimulator of GPR39 activity via the Gα(q), Gα(12/13), and Gα(s) pathways [9394]. Impairments of the central nervous system (CNS), such as defects in learning and memory, may be brought about in the fetus by maternal zinc deficiency, which cannot be reversed by subsequent supplementation of zinc to the diet after weaning [95]. This CNS defect may be associated with a reduction in the number of proliferating stem cells and neuronal precursor cells due to inadequate amount of available zinc [9697]. In various neuronal diseases, such as stroke, epilepsy, mechanical trauma, and Alzheimer's disease, the precise mechanism of cytotoxicity is less known, but emerging evidence suggests that excess Zn2+ may kill neurons through the inhibition of ATP synthesis [98]. In contrast to the previously mentioned proapoptotic role of Zn2+ [84], Zn2+ could participate in the inhibition of proapoptotic caspase-3 activation [71] and in the reduction of amyloid production in experimental neuronal cells [99], since these effects are lost in response to low levels of Zn2+. In zinc deficiency, neural cell apoptosis was associated with an increase in phosphorylation of p53 and its translocation into mitochondria [97].
The extracellular and intracellular levels of Zn2+ may be a determinant to cardiovascular health [29], and zinc deficiency related to diseases and aging may be a factor in the development of heart failure. Moreover, a potential role of zinc in diabetic cardiomyopathy has been suggested, due to its involvement in insulin signaling and diabetes development [100101]. In regards to the cardioprotective roles of Zn2+, four beneficial roles of Zn2+ were suggested: (1) protective effects from acute redox stress in cardiomyocytes; (2) prevention of inflammatory processes triggered during myocardial damage; (3) wound healing; and finally (4) maintenance of cardiac stem cells essential for cardiac healing [29].
In the heart, a large portion of intracellular Zn2+ accumulates in specific cellular structures, such as in or near the terminal sarcoplasmic reticulum (SR) [65]. Calsequestrin is a Zn2+-binding protein residing in the SR and can bind up to 200 mol of Zn2+ per mol [55]. Besides changes in the phosphorylation status of the L-type calcium channel (LTCC), thiol oxidation, and nitrosylation, the extracellular and intracellular levels of Zn2+ influence transmembrane Ca2+ movements via the LTCC. Moreover, intracellular Zn2+ levels could inhibit AC at even low concentrations (<1 nM), which strikingly reduces β-adrenergic stimulation [47]. Moreover, besides being cell permeant, external Zn2+ can prevent Ca2+ entry by inhibiting LTCC at concentrations as low as tens of nanomolars, which in turn suppresses the Ca2+-induced Ca2+ release from the SR [47102]. Zn2+ depresses the contractile force logarithmically with time, which seems to be related with dysregulation in calcium handling in electrically stimulated rat atrial cells [103] or smooth muscle cells [104]. In pre-diabetic rats, the improvement of diastolic relaxation by Zn2+ is attributed to a depressed LTCC inward current, phosphorylation of the ryanodine receptor and phospholamban, resulting in a reduced cellular Ca2+ load [89]. In guinea pigs, the administration of low concentrations of Zn2+ caused mainly sinus bradycardia, while higher concentrations of Zn2+ (30 µM) resulted in various types of arrhythmia that lead to cardiac asystole [105]. In humans, the cardiac ejection fraction increases with Zn2+ content [106], and Zn2+ supplementation to a cardioplegic solution reverses the loss of systolic function [27]. However, the importance of Zn2+ in cardiac muscle function is still incompletely understood.
In patients with ischemic cardiomyopathy, a higher copper level and a lower zinc level were observed [107]. Diabetic patients with congestive heart failure also exhibited a high concentration of urinary Zn2+ excretion [107]. Normally, the majority of plasma Zn2+ is associated with human serum albumin (HSA), but this interaction is allosterically disrupted by free fatty acids (FFAs), which eventually results in hypozincemia due to altered Zn2+ uptake in the cell [21]. Zinc deficiency is associated with enhanced PTP activity, which increases insulin receptor signaling and insulin resistance, which is a risk factor for cardiovascular disease development [108]. Theoretically, all conditions that lead to lower energy consumption by the heart could lead to at least temporary elevations of FFAs. Therefore, the possibility of a direct correlation between lower plasma Zn2+ levels and higher levels of FFAs cannot be overlooked, as it is evident in acute myocardial infarction [109] and chronic heart failure [110]. Zinc deficiency may also have deleterious effects in patients with heart failure, due to decreased intake secondary to decreased absorption, increased urinary loss through diuretics, or high levels of lipid peroxides as a result of oxidative stress [108]. However, paradoxically, heart failure medications, such as thiazide diuretics, angiotensin-converting enzyme inhibitors, angiotensin receptor blockers, and the diuretic furosemide can upset zinc metabolism, and subsequently lead to zinc deficiency [108111].
PKC is a central molecule in many signaling pathways and is particularly prominent in vascular smooth muscle and endothelial cells. As previously mentioned [58], alteration of PKC activity directed by the movement of Zn2+ modulates the action of multiple vasoactive factors that promote atherosclerotic events. Zn2+ may play a protective role in the maintenance of the integrity of endothelial cells [112] or the prevention of ultraviolet-induced skin damage [113]. Chronic zinc deprivation accelerates vascular smooth muscle cell proliferation [114], and in combination with calcification, may aggravate atherosclerosis progression [29].
Pancreatic β cells contain the highest amount of zinc among all cells within the human body. Zn2+ is vital for the precise handling of insulin in β-cells, from storage to the exertion of its effects. It has been suggested that the opening of ATP-sensitive K+ channels (KATP) in the pancreas is regulated by the activation of Zn2+ on both sides of the membrane, with potentially discrete sites of action located on the sulfonylurea receptor (SUR) subunit [115]. It has been recognized that zinc deficiency, or the aberrant expression of ZnT (e.g., ZnT8) or ZIP (e.g., ZIP4) proteins in the pancreas, is linked to the occurrence of pancreatic cancer and chronic pancreatitis [80]. Secreted Zn2+ exhibits autocrine or paracrine signaling in β-cells or neighboring cells. During glucose deprivation, Zn2+ serves as an intercellular signaling ion, and provides an "off-switch" for glucagon release from the pancreatic α-cells. Zn2+ also modulates the exocrine production of digestive enzymes and their role in nutrient absorption. Zinc's entry into the digestive tract is facilitated by enzyme-containing zymogen granules secreted from the acinar cells of the pancreas. The activity of zymogen is Zn2+-dependent, and ZnT2 aids in zinc transport, which eventually leads to digestive enzyme secretion [80].
Unlike other cells, prostate cells contain almost 35% of their total zinc in the cytoplasm, rather than sequester Zn2+ into vesicles and organelles. High zinc levels are emitted into the seminal liquid, assuming a critical role in sperm discharge and motility [116]. Antimicrobial properties have also been associated with Zn2+ in prostatic fluid and the gland itself. In cancer biology, evidence points to zinc's involvement in tumor suppression in several cancers [117]. Reduced levels of zinc in the prostate were also linked to prostate diseases, including benign prostatic hyperplasia and prostate carcinoma [80].
While zinc's role in mammary gland development [80] and remodeling is indisputable, the effects of its deficiency remain poorly understood. A single amino acid substitution in human ZnT2 reduces milk zinc concentration by about 75% and results in severe dermatitis and alopecia in the nursing infant [118]. Tissue expansion in the mammary gland is also mediated by Zn2+-dependent matrix metalloproteinases (MMPs). Again, the availability of cellular Zn2+ dictates the enzyme activity; therefore, a decrease in activity could result in impaired remodeling and subsequent breast disease.
Zinc deficiency is manifested by an immunocompromised status due to thymic atrophy, lymphopenia, or compromised cell- and antibody-mediated responses, which causes increased infection rates with prolonged durations [32119]. Zinc deficiency stimulates the production of cytokines, such as interleukin (IL)-1β, IL-2, IL-6, and tumor necrosis factor (TNF)-α, and the generation of these cytokines shows inverse responses to zinc supplementation [91]. Therefore, mild zinc deficiency with low-grade inflammation has been found in patients with atherosclerosis and diabetes mellitus [91]. In the allergic response, Zn2+ and its transporters are indispensable for Fc epsilon receptor I (FceRI)-mediated mast cell activation, since blocking or supplementation of zinc largely affects this allergic reaction through the involvement of degranulation and cytokine production [12]. In monocytes, Zn2+ affects differentiation by increasing cAMP and its guanosine analog (cGMP) through the inhibition of ACs and cyclic nucleotide phosphodiesterases [46120].
Previous studies have focused on the general maintenance of Zn2+ homeostasis, but recent studies suggest the versatility of Zn2+ as a signaling molecule. As briefly outlined in Fig. 3, numerous functions of tissues, including the brain, immune cells, cardiovascular tissues, pancreas, and prostate and mammary glands, are influenced by the actions of Zn2+. In addition, cancer progression could be associated with the dysregulation of Zn2+ homeostasis and its disturbed cellular signaling. However, the action of Zn2+ is not simple and is heterogeneous in different tissues, as confirmed by the tissue-specific expression of MT, ZnT, and ZIP proteins, and their distribution and possible roles in physiological conditions and disease progression. Cellular redox statuses are also important in understating Zn2+ behavior in a given system, because protein-bound Zn2+, as well as free Zn2+, can be released or bound to certain proteins, thus altering their structure and functions, which ultimately alters cellular responses. Considering the numerous roles of Zn2+ mentioned in this review, Zn2+ and its subsequent signaling events are not simple, and without the regulation of Zn2+, catastrophic responses may occur within cells. This raises the question of how cells can tightly regulate the action of Zn2+, and when it is initiated and terminated. Cellular compartments of Zn2+, regional crosstalk from Zn2+ release or translocation from inside or outside of the cell, and the removal of Zn2+ by MT or other Zn2+-binding proteins may provide a partial explanation as to how Zn2+ is able to partake in several cellular processes. These issues seem to be important, and, thus, more intensive investigation should be undertaken.
As previously mentioned, intracellular Zn2+ levels sensed by MTF-1 can lead to the induction of Zn2+-regulating proteins. However, when cells sustain zinc deficiency, detection mechanisms and the ensuing cellular compensatory processes have not yet been proposed. Therefore, Zn2+ sensing mechanisms in the condition of both excess and deficient levels will be necessary to extend our understanding of the role of Zn2+ in physiology. Except for the known role of MTF-1, there are still gaps in our knowledge of how Zn2+ exerts its action. It should be noted that Zn2+'s role as a signaling molecule and transcriptional regulator may not be solely attributable to Zn2+, since other essential metals are equally important to maintain cellular homeostasis. However, this is beyond the scope of our current review, and the contributions of other ions are not discussed in this manuscript. Inhibition of PTP1B by Zn2+ exposure has been widely recognized. In signal transduction pathways, the phosphorylation status of proteins is regulated by well-known mediators, namely kinases and phosphatases, that influence the functions of proteins. However, direct changes of their activities invoked by the introduction of Zn2+ or the chelation of Zn2+ in proteins are also largely unknown. Therefore, it is also necessary to expand our knowledge of Zn2+'s role as a molecular switch or connector.
Cellular Zn2+ levels can be measured by the aid of Zn2+-specific fluorescent dyes, including cell-impermeable Zinquin-A (free acid), cell permeable Zinquin-E (ethyl ester) [121], TSQ [122], FuraZin-1 AM, RhodZin, and FluoZin. Technically, the movement of Zn2+ is largely determined by Zn2+-specific fluorescent probes. Although these probes are useful in zinc biology, the specificity and sensitivity of Zn2+-detection probes are considered a technical hurdle. Therefore, a rapid and direct method of measurement of Zn2+ should be further developed. A proteomic analysis of Zn2+-containing proteins provides valuable insight into understanding the biological role of Zn2+ [9]. The development of strategies to revert the dysregulation status of cellular Zn2+ will be a promising approach of future direction. In addition, an integrated and systemic understanding of the newly identified roles of Zn2+ will be necessary.
In conclusion, a wider perspective of the roles of Zn2+, beyond being a mere building block of proteins, will be valuable for a comprehensive understanding of the physiological and pathophysiological functions of Zn2+ in the cardiovascular system and other tissues. Further research on the critical roles of Zn2+ and its regulation will aid in the maintenance and improvement of human health.
ACKNOWLEDGMENTS
This work was supported by the National Research Foundation of Korea (NRF), and the funding was granted by the Ministry of Science, ICT & Future Planning of Korea (2012R1A2A1A03007595) and by the Ministry of Education of Korea (2010-0020224).
References
1. Raulin J. Annales des sciences naturelles. Botanique Et BIologie Vegetale. 1869; 11:93–345.
2. Prasad AS, Halsted JA, Nadimi M. Syndrome of iron deficiency anemia, hepatosplenomegaly, hypogonadism, dwarfism and geophagia. Am J Med. 1961; 31:532–546. PMID: 14488490.


3. Maret W, Sandstead HH. Zinc requirements and the risks and benefits of zinc supplementation. J Trace Elem Med Biol. 2006; 20:3–18. PMID: 16632171.


4. Vallee BL, Falchuk KH. The biochemical basis of zinc physiology. Physiol Rev. 1993; 73:79–118. PMID: 8419966.


5. Andreini C, Bertini I, Rosato A. Metalloproteomes: a bioinformatic approach. Acc Chem Res. 2009; 42:1471–1479. PMID: 19697929.


6. Costello LC, Fenselau CC, Franklin RB. Evidence for operation of the direct zinc ligand exchange mechanism for trafficking, transport, and reactivity of zinc in mammalian cells. J Inorg Biochem. 2011; 105:589–599. PMID: 21440525.


7. Dudev T, Lim C. Principles governing Mg, Ca, and Zn binding and selectivity in proteins. Chem Rev. 2003; 103:773–788. PMID: 12630852.


8. Permyakov EA, Kretsinger RH. Cell signaling, beyond cytosolic calcium in eukaryotes. J Inorg Biochem. 2009; 103:77–86. PMID: 18954910.


9. Kochańczyk T, Drozd A, Krężel A. Relationship between the architecture of zinc coordination and zinc binding affinity in proteins--insights into zinc regulation. Metallomics. 2015; 7:244–257. PMID: 25255078.
10. Xu Z, Zhou J. Zinc and myocardial ischemia/reperfusion injury. Biometals. 2013; 26:863–878. PMID: 23982700.


11. Khan MU, Cheema Y, Shahbaz AU, Ahokas RA, Sun Y, Gerling IC, Bhattacharya SK, Weber KT. Mitochondria play a central role in nonischemic cardiomyocyte necrosis: common to acute and chronic stressor states. Pflugers Arch. 2012; 464:123–131. PMID: 22328074.


12. Fukada T, Yamasaki S, Nishida K, Murakami M, Hirano T. Zinc homeostasis and signaling in health and diseases: Zinc signaling. J Biol Inorg Chem. 2011; 16:1123–1134. PMID: 21660546.
13. Oteiza PI. Zinc and the modulation of redox homeostasis. Free Radic Biol Med. 2012; 53:1748–1759. PMID: 22960578.


14. Hughes S, Samman S. The effect of zinc supplementation in humans on plasma lipids, antioxidant status and thrombogenesis. J Am Coll Nutr. 2006; 25:285–291. PMID: 16943449.


15. Taylor KM, Hiscox S, Nicholson RI, Hogstrand C, Kille P. Protein kinase CK2 triggers cytosolic zinc signaling pathways by phosphorylation of zinc channel ZIP7. Sci Signal. 2012; 5:ra11.


17. Takeda A, Nakamura M, Fujii H, Tamano H. Synaptic Zn2+ homeostasis and its significance. Metallomics. 2013; 5:417–423. PMID: 23423555.
18. Maret W. Zinc biochemistry: from a single zinc enzyme to a key element of life. Adv Nutr. 2013; 4:82–91. PMID: 23319127.


19. Cousins RJ. Absorption, transport, and hepatic metabolism of copper and zinc: special reference to metallothionein and ceruloplasmin. Physiol Rev. 1985; 65:238–309. PMID: 3885271.


20. Tran CD, Butler RN, Philcox JC, Rofe AM, Howarth GS, Coyle P. Regional distribution of metallothionein and zinc in the mouse gut: comparison with metallothionien-null mice. Biol Trace Elem Res. 1998; 63:239–251. PMID: 9840820.
21. Barnett JP, Blindauer CA, Kassaar O, Khazaipoul S, Martin EM, Sadler PJ, Stewart AJ. Allosteric modulation of zinc speciation by fatty acids. Biochim Biophys Acta. 2013; 1830:5456–5464. PMID: 23726993.


22. Scott BJ, Bradwell AR. Identification of the serum binding proteins for iron, zinc, cadmium, nickel, and calcium. Clin Chem. 1983; 29:629–633. PMID: 6831689.


23. Taylor KM, Nicholson RI. The LZT proteins; the LIV-1 subfamily of zinc transporters. Biochim Biophys Acta. 2003; 1611:16–30. PMID: 12659941.


24. Taylor KM, Morgan HE, Johnson A, Nicholson RI. Structure-function analysis of a novel member of the LIV-1 subfamily of zinc transporters, ZIP14. FEBS Lett. 2005; 579:427–432. PMID: 15642354.


25. Sekler I, Sensi SL, Hershfinkel M, Silverman WF. Mechanism and regulation of cellular zinc transport. Mol Med. 2007; 13:337–343. PMID: 17622322.


26. Palmiter RD, Huang L. Efflux and compartmentalization of zinc by members of the SLC30 family of solute carriers. Pflugers Arch. 2004; 447:744–751. PMID: 12748859.


27. Powell SR, Aiuto L, Hall D, Tortolani AJ. Zinc supplementation enhances the effectiveness of St. Thomas' Hospital No. 2 cardioplegic solution in an in vitro model of hypothermiccardiac arrest. J Thorac Cardiovasc Surg. 1995; 110:1642–1642. PMID: 8523874.
28. Taylor KM, Morgan HE, Johnson A, Nicholson RI. Structure-function analysis of HKE4, a member of the new LIV-1 subfamily of zinc transporters. Biochem J. 2004; 377:131–139. PMID: 14525538.


29. Little PJ, Bhattacharya R, Moreyra AE, Korichneva IL. Zinc and cardiovascular disease. Nutrition. 2010; 26:1050–1057. PMID: 20950764.


30. Liuzzi JP, Cousins RJ. Mammalian zinc transporters. Annu Rev Nutr. 2004; 24:151–172. PMID: 15189117.


31. Carpenè E, Andreani G, Isani G. Metallothionein functions and structural characteristics. J Trace Elem Med Biol. 2007; 21(Suppl 1):35–39. PMID: 18039494.


32. Coyle P, Philcox JC, Carey LC, Rofe AM. Metallothionein: the multipurpose protein. Cell Mol Life Sci. 2002; 59:627–647. PMID: 12022471.


33. Langmade SJ, Ravindra R, Daniels PJ, Andrews GK. The transcription factor MTF-1 mediates metal regulation of the mouse ZnT1 gene. J Biol Chem. 2000; 275:34803–34809. PMID: 10952993.


34. Masters BA, Quaife CJ, Erickson JC, Kelly EJ, Froelick GJ, Zambrowicz BP, Brinster RL, Palmiter RD. Metallothionein III is expressed in neurons that sequester zinc in synaptic vesicles. J Neurosci. 1994; 14:5844–5857. PMID: 7931547.


35. Colvin RA, Bush AI, Volitakis I, Fontaine CP, Thomas D, Kikuchi K, Holmes WR. Insights into Zn2+ homeostasis in neurons from experimental and modeling studies. Am J Physiol Cell Physiol. 2008; 294:C726–C742. PMID: 18184873.
36. Hogstrand C, Kille P, Nicholson RI, Taylor KM. Zinc transporters and cancer: a potential role for ZIP7 as a hub for tyrosine kinase activation. Trends Mol Med. 2009; 15:101–111. PMID: 19246244.


37. Kiedrowski L. Proton-dependent zinc release from intracellular ligands. J Neurochem. 2014; 130:87–96. PMID: 24606401.


38. Shuttleworth CW, Weiss JH. Zinc: new clues to diverse roles in brain ischemia. Trends Pharmacol Sci. 2011; 32:480–486. PMID: 21621864.


39. Nowakowski A, Petering D. Sensor specific imaging of proteomic Zn2+ with zinquin and TSQ after cellular exposure to N-ethylmaleimide. Metallomics. 2012; 4:448–456. PMID: 22498931.
40. Sensi SL, Ton-That D, Sullivan PG, Jonas EA, Gee KR, Kaczmarek LK, Weiss JH. Modulation of mitochondrial function by endogenous Zn2+ pools. Proc Natl Acad Sci U S A. 2003; 100:6157–6162. PMID: 12724524.
41. Ohana E, Hoch E, Keasar C, Kambe T, Yifrach O, Hershfinkel M, Sekler I. Identification of the Zn2+ binding site and mode of operation of a mammalian Zn2+ transporter. J Biol Chem. 2009; 284:17677–17686. PMID: 19366695.
42. Zou MH, Shi C, Cohen RA. Oxidation of the zinc-thiolate complex and uncoupling of endothelial nitric oxide synthase by peroxynitrite. J Clin Invest. 2002; 109:817–826. PMID: 11901190.


43. Maret W. The function of zinc metallothionein: a link between cellular zinc and redox state. J Nutr. 2000; 130(5S Suppl):1455S–1458S. PMID: 10801959.


44. Kamalov G, Deshmukh PA, Baburyan NY, Gandhi MS, Johnson PL, Ahokas RA, Bhattacharya SK, Sun Y, Gerling IC, Weber KT. Coupled calcium and zinc dyshomeostasis and oxidative stress in cardiac myocytes and mitochondria of rats with chronic aldosteronism. J Cardiovasc Pharmacol. 2009; 53:414–423. PMID: 19333130.
45. Tuncay E, Okatan EN, Toy A, Turan B. Enhancement of cellular antioxidant-defence preserves diastolic dysfunction via regulation of both diastolic Zn2+ and Ca2+ and prevention of RyR2-leak in hyperglycemic cardiomyocytes. Oxid Med Cell Longev. 2014; 2014:290381. PMID: 24693334.
46. Klein C, Sunahara RK, Hudson TY, Heyduk T, Howlett AC. Zinc inhibition of cAMP signaling. J Biol Chem. 2002; 277:11859–11865. PMID: 11805091.


47. Alvarez-Collazo J, Diaz-Garcia CM, Lopez-Medina AI, Vassort G, Alvarez JL. Zinc modulation of basal and β-adrenergically stimulated L-type Ca2+ current in rat ventricular cardiomyocytes: consequences in cardiac diseases. Pflugers Arch. 2012; 464:459–470. PMID: 23007464.
48. Pucéat M, Bony C, Jaconi M, Vassort G. Specific activation of adenylyl cyclase V by a purinergic agonist. FEBS Lett. 1998; 431:189–194. PMID: 9708900.


49. Gómez AM, Kerfant BG, Vassort G. Microtubule disruption modulates Ca2+ signaling in rat cardiac myocytes. Circ Res. 2000; 86:30–36. PMID: 10625302.
50. Volpe SL, Lowe NM, Woodhouse LR, King JC. Effect of maximal exercise on the short-term kinetics of zinc metabolism in sedentary men. Br J Sports Med. 2007; 41:156–161. PMID: 17138634.


51. Bellomo E, Massarotti A, Hogstrand C, Maret W. Zinc ions modulate protein tyrosine phosphatase 1B activity. Metallomics. 2014; 6:1229–1239. PMID: 24793162.


52. Pandey NR, Vardatsikos G, Mehdi MZ, Srivastava AK. Cell-type-specific roles of IGF-1R and EGFR in mediating Zn2+-induced ERK1/2 and PKB phosphorylation. J Biol Inorg Chem. 2010; 15:399–407. PMID: 19946718.
53. Haase H, Maret W. Protein tyrosine phosphatases as targets of the combined insulinomimetic effects of zinc and oxidants. Biometals. 2005; 18:333–338. PMID: 16158225.


54. Aslund F, Beckwith J. Bridge over troubled waters: sensing stress by disulfide bond formation. Cell. 1999; 96:751–753. PMID: 10102262.
55. Turan B, Fliss H, Desilets M. Oxidants increase intracellular free Zn2+ concentration in rabbit ventricular myocytes. Am J Physiol. 1997; 272:H2095–H2106. PMID: 9176274.
56. Tuncay E, Bilginoglu A, Sozmen NN, Zeydanli EN, Ugur M, Vassort G, Turan B. Intracellular free zinc during cardiac excitation-contraction cycle: calcium and redox dependencies. Cardiovasc Res. 2011; 89:634–642. PMID: 21062918.


57. Tuncay E, Okatan EN, Vassort G, Turan B. β-blocker timolol prevents arrhythmogenic Ca2+ release and normalizes Ca2+ and Zn2+ dyshomeostasis in hyperglycemic rat heart. PLoS One. 2013; 8:e71014. PMID: 23923043.
58. Korichneva I, Hoyos B, Chua R, Levi E, Hammerling U. Zinc release from protein kinase C as the common event during activation by lipid second messenger or reactive oxygen. J Biol Chem. 2002; 277:44327–44331. PMID: 12213816.


59. Wang G, Strang C, Pfaffinger PJ, Covarrubias M. Zn2+-dependent redox switch in the intracellular T1-T1 interface of a Kv channel. J Biol Chem. 2007; 282:13637–13647. PMID: 17331952.
60. Zhang S, Kehl SJ, Fedida D. Modulation of Kv1.5 potassium channel gating by extracellular zinc. Biophys J. 2001; 81:125–136. PMID: 11423401.


61. Mahaut-Smith MP. The effect of zinc on calcium and hydrogen ion currents in intact snail neurones. J Exp Biol. 1989; 145:455–464. PMID: 22912993.


62. Stanfield PR. The effect of zinc ions on the gating of the delayed potassium conductance of frog sartorius muscle. J Physiol. 1975; 251:711–735. PMID: 1081141.


63. Vogt K, Mellor J, Tong G, Nicoll R. The actions of synaptically released zinc at hippocampal mossy fiber synapses. Neuron. 2000; 26:187–196. PMID: 10798403.


64. Kerchner GA, Canzoniero LM, Yu SP, Ling C, Choi DW. Zn2+ current is mediated by voltage-gated Ca2+ channels and enhanced by extracellular acidity in mouse cortical neurones. J Physiol. 2000; 528 Pt 1:39–52. PMID: 11018104.
65. Atar D, Backx PH, Appel MM, Gao WD, Marban E. Excitation-transcription coupling mediated by zinc influx through voltage-dependent calcium channels. J Biol Chem. 1995; 270:2473–2477. PMID: 7852308.


66. Andersson DA, Gentry C, Moss S, Bevan S. Clioquinol and pyrithione activate TRPA1 by increasing intracellular Zn2+. Proc Natl Acad Sci U S A. 2009; 106:8374–8379. PMID: 19416844.
67. Hu H, Bandell M, Petrus MJ, Zhu MX, Patapoutian A. Zinc activates damage-sensing TRPA1 ion channels. Nat Chem Biol. 2009; 5:183–190. PMID: 19202543.


68. Dineley KE, Votyakova TV, Reynolds IJ. Zinc inhibition of cellular energy production: implications for mitochondria and neurodegeneration. J Neurochem. 2003; 85:563–570. PMID: 12694382.


69. Ye B, Maret W, Vallee BL. Zinc metallothionein imported into liver mitochondria modulates respiration. Proc Natl Acad Sci U S A. 2001; 98:2317–2322. PMID: 11226237.


70. Kleiner D. The effect of Zn2+ ions on mitochondrial electron transport. Arch Biochem Biophys. 1974; 165:121–125. PMID: 4374126.
71. Maret W, Jacob C, Vallee BL, Fischer EH. Inhibitory sites in enzymes: zinc removal and reactivation by thionein. Proc Natl Acad Sci U S A. 1999; 96:1936–1940. PMID: 10051573.


72. Meeusen JW, Nowakowski A, Petering DH. Reaction of metal-binding ligands with the zinc proteome: zinc sensors and N,N,N',N'-tetrakis(2-pyridylmethyl)ethylenediamine. Inorg Chem. 2012; 51:3625–3632. PMID: 22380934.


73. Vallee BL. Metallothionein: historical review and perspectives. Experientia Suppl. 1979; 34:19–39. PMID: 400521.


74. Maret W, Heffron G, Hill HA, Djuricic D, Jiang LJ, Vallee BL. The ATP/metallothionein interaction: NMR and STM. Biochemistry. 2002; 41:1689–1694. PMID: 11814364.


75. Skulachev VP, Evtodienko IuV, Iasaĭtis AA, Gmirnova EG, Chistiakov VV. Reversible suppression of electron transfer between cytochromes B and C. Vopr Med Khim. 1966; 12:438–440. PMID: 4299500.
76. Berry EA, Zhang Z, Bellamy HD, Huang L. Crystallographic location of two Zn2+-binding sites in the avian cytochrome bc(1) complex. Biochim Biophys Acta. 2000; 1459:440–448. PMID: 11004461.
77. Brown AM, Kristal BS, Effron MS, Shestopalov AI, Ullucci PA, Sheu KF, Blass JP, Cooper AJ. Zn2+ inhibits alpha-ketoglutarate-stimulated mitochondrial respiration and the isolated alpha-ketoglutarate dehydrogenase complex. J Biol Chem. 2000; 275:13441–13447. PMID: 10788456.
78. Gazaryan IG, Krasnikov BF, Ashby GA, Thorneley RN, Kristal BS, Brown AM. Zinc is a potent inhibitor of thiol oxidoreductase activity and stimulates reactive oxygen species production by lipoamide dehydrogenase. J Biol Chem. 2002; 277:10064–10072. PMID: 11744691.


79. Feng W, Cai J, Pierce WM, Franklin RB, Maret W, Benz FW, Kang YJ. Metallothionein transfers zinc to mitochondrial aconitase through a direct interaction in mouse hearts. Biochem Biophys Res Commun. 2005; 332:853–858. PMID: 15913554.


80. Kelleher SL, McCormick NH, Velasquez V, Lopez V. Zinc in specialized secretory tissues: roles in the pancreas, prostate, and mammary gland. Adv Nutr. 2011; 2:101–111. PMID: 22332039.


81. Costello LC, Franklin RB, Feng P. Mitochondrial function, zinc, and intermediary metabolism relationships in normal prostate and prostate cancer. Mitochondrion. 2005; 5:143–153. PMID: 16050980.


82. Devinney MJ, Malaiyandi LM, Vergun O, DeFranco DB, Hastings TG, Dineley KE. A comparison of Zn2+- and Ca2+-triggered depolarization of liver mitochondria reveals no evidence of Zn2+-induced permeability transition. Cell Calcium. 2009; 45:447–455. PMID: 19349076.
83. Wudarczyk J, Debska G, Lenartowicz E. Zinc as an inducer of the membrane permeability transition in rat liver mitochondria. Arch Biochem Biophys. 1999; 363:1–8. PMID: 10049493.


84. Feng P, Li TL, Guan ZX, Franklin RB, Costello LC. Direct effect of zinc on mitochondrial apoptogenesis in prostate cells. Prostate. 2002; 52:311–318. PMID: 12210492.


85. Feng P, Li T, Guan Z, Franklin RB, Costello LC. The involvement of Bax in zinc-induced mitochondrial apoptogenesis in malignant prostate cells. Mol Cancer. 2008; 7:25. PMID: 18331646.


86. Calderone A, Jover T, Mashiko T, Noh KM, Tanaka H, Bennett MV, Zukin RS. Late calcium EDTA rescues hippocampal CA1 neurons from global ischemia-induced death. J Neurosci. 2004; 24:9903–9913. PMID: 15525775.


87. McCord MC, Aizenman E. The role of intracellular zinc release in aging, oxidative stress, and Alzheimer's disease. Front Aging Neurosci. 2014; 6:77. PMID: 24860495.


88. Haase H, Rink L. Multiple impacts of zinc on immune function. Metallomics. 2014; 6:1175–1180. PMID: 24531756.


90. Frederickson C, Frederickson CJ, Maret W, Sandstead H, Giblin L, Thompson R. Meeting report: zinc signals 2007-expanding roles of the free zinc ion in biology. Sci STKE. 2007; 2007:pe61. PMID: 17986712.


91. Foster M, Samman S. Zinc and regulation of inflammatory cytokines: implications for cardiometabolic disease. Nutrients. 2012; 4:676–694. PMID: 22852057.


92. Holst B, Egerod KL, Schild E, Vickers SP, Cheetham S, Gerlach LO, Storjohann L, Stidsen CE, Jones R, Beck-Sickinger AG, Schwartz TW. GPR39 signaling is stimulated by zinc ions but not by obestatin. Endocrinology. 2007; 148:13–20. PMID: 16959833.


93. Młyniec K, Budziszewska B, Holst B, Ostachowicz B, Nowak G. GPR39 (zinc receptor) knockout mice exhibit depressionlike behavior and CREB/BDNF down-regulation in the hippocampus. Int J Neuropsychopharmacol. 2014; 18:pii: pyu002. DOI: 10.1093/ijnp/pyu002.


94. Popovics P, Stewart AJ. GPR39: a Zn2+-activated G protein-coupled receptor that regulates pancreatic, gastrointestinal and neuronal functions. Cell Mol Life Sci. 2011; 68:85–95. PMID: 20812023.
95. Halas ES, Hunt CD, Eberhardt MJ. Learning and memory disabilities in young adult rats from mildly zinc deficient dams. Physiol Behav. 1986; 37:451–458. PMID: 3749304.


96. Adamo AM, Zago MP, Mackenzie GG, Aimo L, Keen CL, Keenan A, Oteiza PI. The role of zinc in the modulation of neuronal proliferation and apoptosis. Neurotox Res. 2010; 17:1–14. PMID: 19784710.


97. Seth R, Corniola RS, Gower-Winter SD, Morgan TJ Jr, Bishop B, Levenson CW. Zinc deficiency induces apoptosis via mitochondrial p53- and caspase-dependent pathways in human neuronal precursor cells. J Trace Elem Med Biol. 2015; 30:59–65. PMID: 25467851.


98. Weiss JH, Sensi SL, Koh JY. Zn2+: a novel ionic mediator of neural injury in brain disease. Trends Pharmacol Sci. 2000; 21:395–401. PMID: 11050320.
99. Lee J, Kim CH, Kim DG, Ahn YS. Zinc inhibits amyloid beta production from Alzheimer's amyloid precursor protein in SH-SY5Y cells. Korean J Physiol Pharmacol. 2009; 13:195–200. PMID: 19885037.
100. Tamaki M, Fujitani Y. Role of zinc in type 2 diabetes. Nihon Eiseigaku Zasshi. 2014; 69:15–23. PMID: 24476591.


101. Miao X, Sun W, Fu Y, Miao L, Cai L. Zinc homeostasis in the metabolic syndrome and diabetes. Front Med. 2013; 7:31–52. PMID: 23385610.


102. Yi T, Vick JS, Vecchio MJ, Begin KJ, Bell SP, Delay RJ, Palmer BM. Identifying cellular mechanisms of zinc-induced relaxation in isolated cardiomyocytes. Am J Physiol Heart Circ Physiol. 2013; 305:H706–H715. PMID: 23812383.


103. Ciofalo FR, Thomas LJ Jr. The effects of zinc on contractility, membrane potentials, and cation content of rat atria. J Gen Physiol. 1965; 48:825–839. PMID: 14324990.


104. Schnieden H, Small RC. Spasmolytic effects of cadmium and zinc ions upon the guinea-pig isolated ileum preparation. Br J Pharmacol. 1971; 41:488–499. PMID: 4396970.


105. Evangelou A, Kalfakakou V. Electrocardiographic alterations induced by zinc ions on isolated guinea pig heart preparations. Biol Trace Elem Res. 1993; 36:203–208. PMID: 7681312.


106. Oster O, Dahm M, Oelert H. Element concentrations (selenium, copper, zinc, iron, magnesium, potassium, phosphorous) in heart tissue of patients with coronary heart disease correlated with physiological parameters of the heart. Eur Heart J. 1993; 14:770–774. PMID: 8325303.


107. Shokrzadeh M, Ghaemian A, Salehifar E, Aliakbari S, Saravi SS, Ebrahimi P. Serum zinc and copper levels in ischemic cardiomyopathy. Biol Trace Elem Res. 2009; 127:116–123. PMID: 18953508.


108. Witte KK, Clark AL, Cleland JG. Chronic heart failure and micronutrients. J Am Coll Cardiol. 2001; 37:1765–1774. PMID: 11401109.


109. Gomez E, del Diego C, Orden I, Elósegui LM, Borque L, Escanero JF. Longitudinal study of serum copper and zinc levels and their distribution in blood proteins after acute myocardial infarction. J Trace Elem Med Biol. 2000; 14:65–70. PMID: 10941714.


110. Foster M, Samman S. Zinc and redox signaling: perturbations associated with cardiovascular disease and diabetes mellitus. Antioxid Redox Signal. 2010; 13:1549–1573. PMID: 20568953.


111. Cohen N, Golik A. Zinc balance and medications commonly used in the management of heart failure. Heart Fail Rev. 2006; 11:19–24. PMID: 16819574.


112. Meerarani P, Reiterer G, Toborek M, Hennig B. Zinc modulates PPARgamma signaling and activation of porcine endothelial cells. J Nutr. 2003; 133:3058–3064. PMID: 14519784.
113. Cho YS, Lee KH, Park JW. Pyrithione-zinc Prevents UVB-induced Epidermal Hyperplasia by Inducing HIF-1alpha. Korean J Physiol Pharmacol. 2010; 14:91–97. PMID: 20473380.
114. Alcantara EH, Shin MY, Feldmann J, Nixon GF, Beattie JH, Kwun IS. Long-term zinc deprivation accelerates rat vascular smooth muscle cell proliferation involving the down-regulation of JNK1/2 expression in MAPK signaling. Atherosclerosis. 2013; 228:46–52. PMID: 23466072.


115. Prost AL, Bloc A, Hussy N, Derand R, Vivaudou M. Zinc is both an intracellular and extracellular regulator of KATP channel function. J Physiol. 2004; 559:157–167. PMID: 15218066.
116. Wroblewski N, Schill WB, Henkel R. Metal chelators change the human sperm motility pattern. Fertil Steril. 2003; 79(Suppl 3):1584–1589. PMID: 12801564.


117. Costello LC, Franklin RB. Cytotoxic/tumor suppressor role of zinc for the treatment of cancer: an enigma and an opportunity. Expert Rev Anticancer Ther. 2012; 12:121–128. PMID: 22149438.


118. Chowanadisai W, Lönnerdal B, Kelleher SL. Identification of a mutation in SLC30A2 (ZnT-2) in women with low milk zinc concentration that results in transient neonatal zinc deficiency. J Biol Chem. 2006; 281:39699–39707. PMID: 17065149.


119. Fraker PJ, King LE. Reprogramming of the immune system during zinc deficiency. Annu Rev Nutr. 2004; 24:277–298. PMID: 15189122.


120. Donnelly TE Jr. Effects of zinc chloride on the hydrolysis of cyclic GMP and cyclic AMP by the activator-dependent cyclic nucleotide phosphodiesterase from bovine heart. Biochim Biophys Acta. 1978; 522:151–160. PMID: 202321.


121. Haase H, Beyersmann D. Intracellular zinc distribution and transport in C6 rat glioma cells. Biochem Biophys Res Commun. 2002; 296:923–928. PMID: 12200136.


122. Frederickson CJ, Kasarskis EJ, Ringo D, Frederickson RE. A quinoline fluorescence method for visualizing and assaying the histochemically reactive zinc (bouton zinc) in the brain. J Neurosci Methods. 1987; 20:91–103. PMID: 3600033.


Fig. 1
Impact of Zn2+ concentration on protein function in the cell. The total zinc concentration in a eukaryotic cell is relatively high (100~300 µM), but the actual amount of Zn2+ ranges from ~pM to ~nM values, depending on the cell type. Both extremely low and extremely high levels of Zn2+ have adverse effects on the cell. Excess Zn2+ causes irreversible effects on proteins, such as aggregation, which leads to the dysfunction of many proteins. Low levels of Zn2+ are also detrimental to the cell, since it is an important metal cofactor and signal transducer.
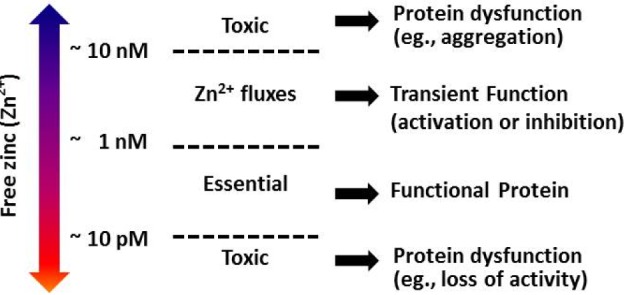
Fig. 2
Subcellular localization of Zn2+ transporters. Zinc absorbed in the intestine as an ion goes into the blood stream. Serum albumin is a major Zn2+ binding protein and serves as a macro Zn2+ transporter to target tissues; thus, changes in the concentration of albumin or an increase of fatty acids, which interferes with Zn2+ binding to albumin, impairs the control of blood Zn2+ levels. The availability of intracellular Zn2+ is governed by the action of MTs (MTs; binding or trapping of Zn2+), zinc transporters (ZnTs; Zn2+ efflux), Zn2+ importers (ZIPs; Zn2+ influx) residing in the plasma membrane, redox potential, pH, and other unknown modulators. ZIPs and ZnTs residing in the organelle membrane have an inverse direction compared to those located in the plasma membrane. The activation of metalresponsive-element-binding transcription factor-1 (MTF-1) increases the expression of MTs, and thereby reduces Zn2+ levels upon binding to Zn2+. Specific forms of MTs, ZnTs, and ZIPs are dependent on cell-type, which will give varying responses, even at the same zinc levels. Extracellular Zn2+ entering the cell could be immediately buffered within the still undefined 'zinc muffler' and translocated into an intracellular Zn2+ store, such as the endoplasmic reticulum (ER) and Golgi apparatus by ZnT. Inversely, Zn2+ released into the cytosol from Zn2+ stores by ZIPs is also buffered by a cytosolic Zn2+ muffler. Arrows show the predicted direction of Zn2+ mobilization.
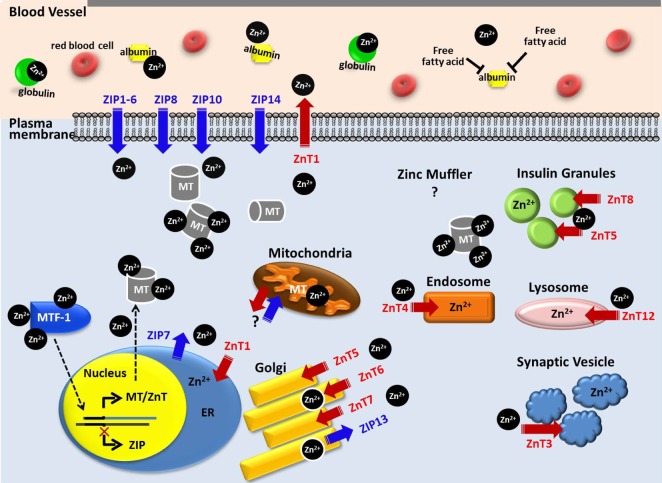
Fig. 3
Roles of Zn2+ in a biological system. Various tissues and organs are influenced by Zn2+. The intracellular Zn2+ levels are primarily regulated by MTs, ZnTs, and ZIPs. The action of Zn2+ can be initiated within a few minutes by protein modification involved in enzyme activities and signal transduction, or in late events, which are mediated by gene regulation. Therefore, the impairment of Zn2+ homeostasis, such as deficiency or excess, can lead to cellular malfunction, and eventually, cell damage.
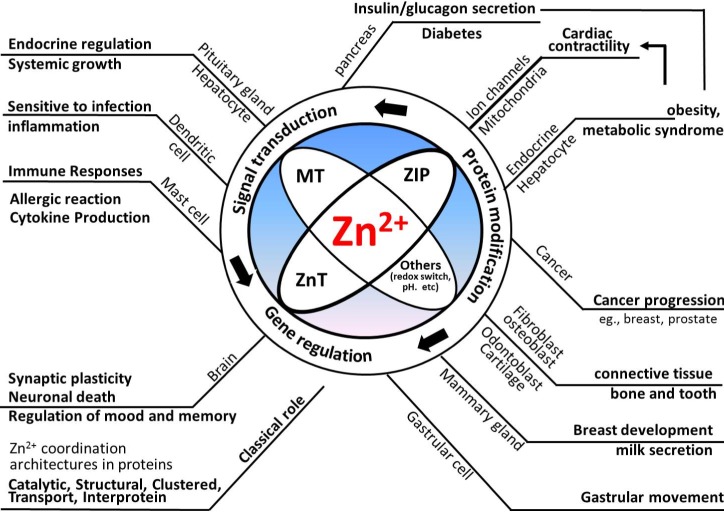