Abstract
Under some pathological conditions as bile flow obstruction or liver diseases with the enterohepatic circulation being disrupted, regurgitation of bile acids into the systemic circulation occurs and the plasma level of bile acids increases. Bile acids in circulation may affect the nervous system. We examined this possibility by studying the effects of bile acids on gating of neuronal (N)-type Ca2+ channel that is essential for neurotransmitter release at synapses of the peripheral and central nervous system. N-type Ca2+ channel currents were recorded from bullfrog sympathetic neuron under a cell-attached mode using 100 mM Ba2+ as a charge carrier. Cholic acid (CA, 10-6 M) that is relatively hydrophilic thus less cytotoxic was included in the pipette solution. CA suppressed the open probability of N-type Ca2+ channel, which appeared to be due to an increase in null (no activity) sweeps. For example, the proportion of null sweep in the presence of CA was ~40% at +40 mV as compared with ~8% in the control recorded without CA. Other single channel properties including slope conductance, single channel current amplitude, open and shut times were not significantly affected by CA being present. The results suggest that CA could modulate N-type Ca2+ channel gating at a concentration as low as 10-6 M. Bile acids have been shown to activate nonselective cation conductance and depolarize the cell membrane. Under pathological conditions with increased circulating bile acids, CA suppression of N-type Ca2+ channel function may be beneficial against overexcitation of the synapses.
Bile acids are formed from cholesterol in the hepatocyte and stored in gallbladder, being released for transport lipids as mixed micells in the small intestine thereby promoting lipid absorption [1]. In health, the enterohepatic circulation efficiently conserves bile acids, which results in the concentration of bile acids in plasma being extremely low [2]. However, under pathological conditions as bile flow obstruction or bile duct disease, regurgitation of bile acids into the systemic blood stream occurs, resulting in an increased plasma level of bile acids as high as to 500~600 µm [3]. Increase in circulating level of bile acids may lead to a wide variety of pathophysiological conditions [4,5].
Physiological role of bile acids besides emulsifying lipids have been recognized, for example, in glucose homeostasis [6-8], thyroid function [9], and cardiovascular function [10]. Bile acids also could produce PGE2 via activation of COX-2 [11], and directly interact with muscarinic receptors [12]. These actions of bile acids appear to be mediated through their binding to specific receptors. Recently, an existence of cell surface receptors [13,14] besides nuclear receptors [15-17] has been proposed, which is thought to be coupled with G-protein [14,18,19]. Bile acids also may directly activate ion channel protein such as large conductance Ca2+ activated K+ channel [20].
Little information is available on the effects of bile acids on the nervous system. High concentration of bile acids free in circulation may affect the function of peripheral and/or central neurons. In the present study, we explored this possibility by studying the effects of bile acids on neuronal (N)-type Ca2+ channel that is known to be essential for neurotransmitter release at synapses of the peripheral and central nervous system [21]. Biophysical properties of N-type Ca2+ channel at a single channel level have been extensively characterized in bullfrog sympathetic neuron [22], in which a major proportion of functionally expressed Ca2+ channel is N-type. Therefore, this system was adopted to assess the effects of low concentration of cholic acid (CA) that is relatively hydrophilic [23,24] thus less damaging to the cell membrane or least cytotoxic [25].
Neurons were isolated from caudal paravertebral sympathetic ganglia of adult bull frogs (Rana Catesbeiana) and dissociated by a collagenase/dispase digestion and trituration [22]. Cells were maintained in L-15 culture medium supplemented with 10% fetal bovine serum and penicillin/streptomycin. Cells were stored at 4℃ until use.
N-type Ca2+ channel currents were recorded under a cell attached mode at room temperature using 100 mM Ba2+ as a charge carrier. The pipette solution contained (in mM): 100 BaCl2, 10 tetraethylammonium chloride, 5 4-aminopyridine and 10 N-methyl-D-glucamine (NMG)-HEPES. The extracellular solution was designed to zero the cell's membrane potential and contained (in mM) 100 KCl, 10 KHEPES, and 5 NMG-EGTA, pH 7.2. The zeroing solution contained 10-6 M (±) Bay K8644 to reveal the presence of L-type Ca2+ channels in the patch. Patches containing L-type Ca2+ channels were excluded from analysis.
Electrodes for single channel experiments were fabricated from Corning 7052 (o.d. 1.5 mm, i.d. 0.86 mm: A-M Systems, Sequim, WA) glass. These electrodes had resistances ranging from 15 to 40 MΩ, which were coated with Sylgard (GE Silicones RTV615; General Electric Co.).
The Voltage clamp test pulse protocols were delivered, and single channel current responses were amplified and filtered (at 2 kHz) by means of a patch clamp amplifier (Axopatch 200B, Axon Instruments, Foster City, CA) interfaced to a MacAdios II analogue-digital converter (GW Instruments) and a Macintosh computer running S3 data acquisition software written by Dr. Stephen Ikeda (NIH, Rockville, MA). Currents were amplified an additional 10 times by a Bessel filter (Warner, LPF-100B). Data were obtained in sets of 100 voltage steps of 100 ms duration delivered at a 2s interval, and analyzed using IgorPro software (Wavemetrics).
Cholic acid (CA) at 10-6 M inhibited N-type Ca2+ channel currents. CA increased the frequency of null (no activity) sweeps resulting a decrease in amplitude of pseudomacroscopic currents (Fig. 1). The proportion of null sweeps in the presence of CA in this specific patch was 71, 46 and 40% at +20, +30 and +40 mV, respectively, whereas they were 15, 12 and 8 in the control patch recorded without CA. When active sweeps are compared in diary plots (bottom trace), the channels from the two patches were gating with similar open probability (Po).
An increase in the frequency of null by CA was manifested in a decreased Po of the channel (Fig. 2). The Po was calculated from a 100 sweep data set including null sweeps and averaged over several patches. For example, at +30 mV the Po was 0.16±0.04 (n=3 patches/3 frogs) in the presence of CA (closed circle), while that was 0.36±0.05 (n=4 patches/3 frogs) in the control without CA (open circle). The data were fit by a single Boltzmann equation. In the presence of CA, the Boltzmann half activation voltage (V1/2) was 30 mV and a slope of e fold change was 8 mV with maximum Po of 0.3, while in the control without CA they were 30 mV, 6 mV and 0.6, respectively.
The effects of CA on other biophysical properties of N-type Ca2+ channel was also determined (Fig. 3). In the presence of CA (10-6 M) the single channel current amplitude appeared to be similar to that of control (Fig. 3A), suggesting that channel permeation was not affected by CA. The instantaneous current-voltage relationship seemed to be also unaffected by CA (Fig. 3B). In the presence of CA, the current elicited by a ramp voltage from -80 to +120 mV (ramp speed of 1 mV/ms) was resolvable at potentials positive to ~+10 mV and the mean voltages generating the peak current was ~+35 mV (n=5 patches/3 frogs, Fig. 3B), which is similar to that of control patches (data not shown). Single channel current amplitude measured at each patch potential was averaged from 5 patches and plotted against patch potential (Fig. 3C). The slope conductance was calculated from 0 to +50 mV by linear regression and was found to be 21.2±1.7 pS with a single channel current at 0 mV of -1.3±0.1 pA (n=5 patches/3 frogs, Fig. 3C), which were almost identical to those of controls and the properties of N-type Ca2+ channel previously reported [22].
Mean open time and shut time histograms of N type Ca2+ channel in the presence of CA are illustrated in Fig. 4. The open time histograms were well fit by a single exponential. The mean open time (τo) increased with voltage (n=5 patches/3 frogs, containing one or two channels). The shut time histograms were fit by two exponentials with the longer component (τsh2) decreased with voltage (n=3 patches/3 frogs, containing one channel). These properties are consistent with those of N-type Ca2+ channel [22]. For example, at +30 mV the mean open time was 1.6 ms and the mean shut times were 0.4 and 2.6 ms in the presence of CA, which were similar to those of controls (1.4 ms, and 0.4 and 2.5 ms, respectively). Thus, CA did not seem to affect the duration of N-type Ca2+ channel opening and closing.
The present study demonstrates that CA inhibits N-type Ca2+ channel function by altering channel gating. Other single channel properties such as current-voltage relationship, unitary slope conductance, single channel current amplitude, open times and shut times were unaffected by CA. CA increased the frequency of null (Figs. 1 and 3) and consequently decreased Po. The null sweeps appeared to be clustered, suggesting that an open state is not attainable for a period of time during which the channel may not be responsive to excitatory signals or depolarization. Thus, under pathological conditions where circulating bile acids get elevated, N-type Ca2+ channel function may be suppressed leading to a synaptic depression.
The mechanism by which CA modulates N-type Ca2+ channel is obscure. It is not due to cytotoxicity, since CA is one of the least cytotoxic bile acids [25]. Nonspecific membrane damage and cell lysis [26] is unlikely, since the concentration used in the present experiment is at least 1,000 times less than the concentration producing membrane toxicity [27] or critical micelle concentration [28-30].
One possibility is that CA may modulate N-type Ca2+ channel function by directly interacting with channel protein. However, one can argue that binding of bile acids to protein is correlated with their hydrophobicity and CA is relatively hydrophilic among bile acids [23,24]. Dissociation constant of CA to albumin was 333 µM [31-33] that is ~300 times greater than the concentration used in the present study. Thus, suppression of Ca2+ channel in the presence of 1 µM CA may not be entirely attributable to CA binding directly to the channel protein.
Another possibility is that CA may act through a G-protein coupled cell surface receptor protein [14]. G protein βγ subunit has been shown to modulate N-type Ca2+ channel gating in a membrane delimited manner [34]. However, in the presence of norepinephrine known to inhibit N-type Ca2+ channel gating through G protein βγ subunit, no significant increase in null sweeps was observed [35], ruling out the possibility of involving membrane delimited pathway.
Finally, CA may alter local lipid invironement where the channel protein is situated. Synthetic detergent Triton-X100 (10-5 M) that decreases bilayer stiffness or promotes convex curvature of the membrane lipid shifted steady state voltage-dependent inactivation of N-type Ca2+ channel toward more negative potentials [36]. CA, due to its amphipatic nature, may also affect local lipid environment similarly and modify inactivation property of N-type Ca2+ channel. One mechanism generating null sweeps may be inactivation from intermediate closed states of the channel on its way to opening [22,37,38]. If that is true, an increase in null sweeps and consequent rapid inactivation will lead to a left shift of the inactivation-voltage relationship. However, we did not see any significant increase in null sweeps by Triton-X100 at 10-6 M under the identical experimental condition (data not shown). Therefore, the possibility of involving a specific protein effecting the interaction of CA on N-type Ca2+ channel gating still remains valid.
N-type Ca2+ channel is one of the main Ca2+ channels known to mediate neurotransmitter release at presynaptic terminals [39,40]. N-type Ca2+ channel is required for hippocampus-dependent learning and memory [41], sensory transmission [42,43], pain transduction in particular [44] and autonomic function. In mice deficient of N-type Ca2+ channel exhibited a substantial reduction in basal synaptic transmission [41], higher pain threshold [45] and decreased sympathetic nerve activity [46]. Thus, it is reasonable to predict that a high concentration of circulating CA, by suppressing N-type Ca2+ channel function, could result in similar pathophysiological effects, but to different levels. Several studies have shown in various cell types that bile acids activated nonselective cation conductance (INSC). Taurodeoxycholic acid, dexoycholic acid or diluted fresh bile have been shown to activate INSC [47,48] and/or Na+-dependent membrane depolarization [48,49]. Under some pathological conditions with increased concentrations of bile acids, the suppression of N-type Ca2+ channel function by CA may be beneficial in moderating excitation of the synapses.
Figures and Tables
Fig. 1
Example traces for typical effects of cholic acid (CA) on single N-type Ca2+ channel activity at +40 mV. (A) Twelve consecutive sweeps are shown from a single N-type Ca2+ channel patch recorded in the absence of CA. Data were obtained by applying 100 ms voltage steps every 2 sec. Pseudomacroscopic current below the sweeps was averaged from 100 sweeps. A diary plot of open probability (Po) measured over the 100 ms sweep duration is shown at the bottom for 100 consecutive voltage steps to +40 mV from holding potential of -40 mV. The brackets illustrate the areas shown in the trace above. (B) Recordings from another single channel patch in the presence of CA (10-6 M) applied in the pipette solution. Note an increase in null (no activity) sweeps with decreased pseudomacroscopic current. Diary plot indicates similar Po gating in active sweeps between the two patches. The illustrated records were Gaussian filtered at 1 kHz.
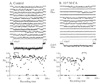
Fig. 2
Effects of cholic acid (CA) on open probability (Po) of N-type Ca2+ channel measured from patches containing a single N-type Ca2+ channel. CA (10-6 M) suppressed Po measured over 100-ms voltage steps to various voltages from holding potential of -40 mV. Each symbol represents the mean Po (included nulls) calculated from a 100 sweep data set and averaged over several patches. Open circles represent the patch recorded without CA (n=4 patches/3 frogs) and closed circles are from the patch recorded with CA included in the pipette solution (n=3 patches/3 frogs). The solid (CA) and dashed (control) lines are from a single Boltzmann fit to all points. The parameters for the line are V1/2=30 mV, slope=e-fold for 8 mV, and maximum Po=0.3 for CA, and V1/2=30 mV, slope=e-fold for 6 mV, and maximum Po=0.6 for control. *p<0.05.
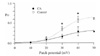
Fig. 3
Effects of cholic acid (CA) on other biophysical properties of N-type Ca2+ channel. (A) Twelve consecutive sweeps recorded in the presence of CA (10-6 M) are shown for each voltage. This patch contained a single N-type Ca2+ channel. (B) Instantaneous current-voltage relationship from the patch illustrated in A. The ramp current elicited by a ramp voltage from -120 to +80 mV (ramp speed 1 mV/ms) was averaged from 10 sweeps. (C) The slope conductance (γ) was calculated by linear regression and was 21.2±1.7 pS and a singe channel current at 0 mV of 1.3±0.1 pA (n=5 patches/3 frogs), which were not different from those of control patches or those of typical N-type Ca2+ channel.
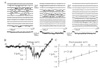
Fig. 4
Effects of cholic acid (CA) on mean open and shut times of N-type Ca2+ channel. (A) The open time histograms measured from five patches containing one or two N-type Ca2+ channel were fit by a single exponential. The mean open time (τo) increased with voltage (+20, +30, +40 mV from the top to the bottom), which were similar to those of controls. (B) The shut time histograms measured from three patches containing a single N-type Ca2+ channel were fit by two exponentials. The brief component (τsh1) did not vary with voltage, while the longer component (τsh2) decreased with voltage. These properties were similar to those of controls and those of typical N-type Ca2+ channel.
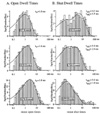
ACKNOWLEDGEMENTS
This work was supported by a grant (03-316) from the Asan Institute for Life Sciences, Seoul, Korea, and the grant No. R05-2000-000-00190-0 from the Basic Research Program of the Korea Science & Engineering Foundation.
References
1. Borgstrom B, Barrowman JA, Lindstrom M. Danielsson H, Sjovall J, editors. Roles of bile acids in intestinal lipid digestion in absorption. Sterols and bile acids. 1985. Amsterdam: Elsevir;405–425.
2. Hofmann AF. Chemistry and enterohepatic circulation of bile acids. Hepatology. 1984. 4:5 Suppl. 4S–14S.
3. Osuga T, Mitamura K, Mashige F, Imai D. Evaluation of fluorimetrically estimated serum bile acid in liver disease. Clin Chim Acta. 1977. 75:81–90.
4. Hofmann AF. Bile acids: the good, the bad, and the ugly. News Physiol Sci. 1999. 14:24–29.
5. Shah N, Khurana S, Cheng K, Raufman JP. Muscarinic receptors and ligands in cancer. Am J Physiol Cell Physiol. 2009. 296:C221–C232.
6. Patti ME, Houten SM, Bianco AC, Bernier R, Larsen PR, Holst JJ, Badman MK, Maratos-Flier E, Mun EC, Pihlajamaki J, Auwerx J, Goldfine AB. Serum bile acids are higher in humans with prior gastric bypass: potential contribution to improved glucose and lipid metabolism. Obesity (Silver Spring). 2009. 17:1671–1677.
7. Thomas C, Gioiello A, Noriega L, Strehle A, Oury J, Rizzo G, Macchiarulo A, Yamamoto H, Mataki C, Pruzanski M, Pellicciari R, Auwerx J, Schoonjans K. TGR5-mediated bile acid sensing controls glucose homeostasis. Cell Metab. 2009. 10:167–177.
8. Jansen PL. A new life for bile acids. J Hepatol. 2010. 52:937–938.
9. Thomas C, Auwerx J, Schoonjans K. Bile acids and the membrane bile acid receptor TGR5--connecting nutrition and metabolism. Thyroid. 2008. 18:167–174.
10. Khurana S, Raufman JP, Pallone TL. Bile acids regulate cardiovascular function. Clin Transl Sci. 2011. 4:210–218.
11. Jun JY, Choi S, Chang IY, Yoon CK, Jeong HG, Kong ID, So I, Kim KW, You HJ. Deoxycholic acid inhibits pacemaker currents by activating ATP-dependent K+ channels through prostaglandin E2 in interstitial cells of Cajal from the murine small intestine. Br J Pharmacol. 2005. 144:242–251.
12. Raufman JP, Cheng K, Zimniak P. Activation of muscarinic receptor signaling by bile acids: physiological and medical implications. Dig Dis Sci. 2003. 48:1431–1444.
13. Maruyama T, Miyamoto Y, Nakamura T, Tamai Y, Okada H, Sugiyama E, Nakamura T, Itadani H, Tanaka K. Identification of membrane-type receptor for bile acids (M-BAR). Biochem Biophys Res Commun. 2002. 298:714–719.
14. Kawamata Y, Fujii R, Hosoya M, Harada M, Yoshida H, Miwa M, Fukusumi S, Habata Y, Itoh T, Shintani Y, Hinuma S, Fujisawa Y, Fujino M. A G protein-coupled receptor responsive to bile acids. J Biol Chem. 2003. 278:9435–9440.
15. Makishima M, Okamoto AY, Repa JJ, Tu H, Learned RM, Luk A, Hull MV, Lustig KD, Mangelsdorf DJ, Shan B. Identification of a nuclear receptor for bile acids. Science. 1999. 284:1362–1365.
16. Zimber A, Gespach C. Bile acids and derivatives, their nuclear receptors FXR, PXR and ligands: role in health and disease and their therapeutic potential. Anticancer Agents Med Chem. 2008. 8:540–563.
17. Hylemon PB, Zhou H, Pandak WM, Ren S, Gil G, Dent P. Bile acids as regulatory molecules. . J Lipid Res. 2009. 50:1509–1520.
18. Kostenis E. A glance at G-protein-coupled receptors for lipid mediators: a growing receptor family with remarkably diverse ligands. Pharmacol Ther. 2004. 102:243–257.
19. Tiwari A, Maiti P. TGR5: an emerging bile acid G-protein-coupled receptor target for the potential treatment of metabolic disorders. Drug Discov Today. 2009. 14:523–530.
20. Dopico AM, Walsh JV Jr, Singer JJ. Natural bile acids and synthetic analogues modulate large conductance Ca2+-activated K+ (BKCa) channel activity in smooth muscle cells. J Gen Physiol. 2002. 119:251–273.
21. Hirning LD, Fox AP, McCleskey EW, Olivera BM, Thayer SA, Miller RJ, Tsien RW. Dominant role of N-type Ca2+ channels in evoked release of norepinephrine from sympathetic neurons. Science. 1988. 239:57–61.
22. Lee HK, Elmslie KS. Gating of single N-type calcium channels recorded from bullfrog sympathetic neurons. J Gen Physiol. 1999. 113:111–124.
23. Heuman DM. Quantitative estimation of the hydrophilichydrophobic balance of mixed bile salt solutions. J Lipid Res. 1989. 30:719–730.
24. Bomzon A, Ljubuncic P. Bile acids as endogenous vasodilators? Biochem Pharmacol. 1995. 49:581–589.
25. Sharma R, Majer F, Peta VK, Wang J, Keaveney R, Kelleher D, Long A, Gilmer JF. Bile acid toxicity structure-activity relationships: correlations between cell viability and lipophilicity in a panel of new and known bile acids using an oesophageal cell line (HET-1A). Bioorg Med Chem. 2010. 18:6886–6895.
26. Weltzien HU. Cytolytic and membrane-perturbing properties of lysophosphatidylcholine. Biochim Biophys Acta. 1979. 559:259–287.
27. Posa M, Kuhajda K. Hydrophobicity and haemolytic potential of oxo derivatives of cholic, deoxycholic and chenodeoxycholic acids. Steroids. 2010. 75:424–431.
28. Roda A, Hofmann AF, Mysels KJ. The influence of bile salt structure on self-association in aqueous solutions. J Biol Chem. 1983. 258:6362–6370.
29. Posa M, Kevresan S, Mikov M, Cirin-Novta V, Sârbu C, Kuhajda K. Determination of critical micellar concentrations of cholic acid and its keto derivatives. Colloids Surf B Biointerfaces. 2007. 59:179–183.
30. Yang L, Zhang H, Mikov M, Tucker IG. Physicochemical and biological characterization of monoketocholic acid, a novel permeability enhancer. Mol Pharm. 2009. 6:448–456.
31. Roda A, Cappelleri G, Aldini R, Roda E, Barbara L. Quantitative aspects of the interaction of bile acids with human serum albumin. J Lipid Res. 1982. 23:490–495.
32. Pico GA, Houssier C. Bile salts-bovine serum albumin binding: spectroscopic and thermodynamic studies. Biochim Biophys Acta. 1989. 999:128–134.
33. Ceryak S, Bouscarel B, Fromm H. Comparative binding of bile acids to serum lipoproteins and albumin. J Lipid Res. 1993. 34:1661–1674.
34. Ikeda SR. Voltage-dependent modulation of N-type calcium channels by G-protein beta gamma subunits. Nature. 1996. 380:255–258.
35. Lee HK, Elmslie KS. Reluctant gating of single N-type calcium channels during neurotransmitter-induced inhibition in bullfrog sympathetic neurons. J Neurosci. 2000. 20:3115–3128.
36. Lundbaek JA, Birn P, Girshman J, Hansen AJ, Andersen OS. Membrane stiffness and channel function. Biochemistry. 1996. 35:3825–3830.
37. Klemic KG, Shieh CC, Kirsch GE, Jones SW. Inactivation of Kv2.1 potassium channels. Biophys J. 1998. 74:1779–1789.
38. Patil PG, Brody DL, Yue DT. Preferential closed-state inactivation of neuronal calcium channels. Neuron. 1998. 20:1027–1038.
39. Dunlap K, Luebke JI, Turner TJ. Exocytotic Ca2+ channels in mammalian central neurons. Trends Neurosci. 1995. 18:89–98.
40. Wu LG, Saggau P. Presynaptic inhibition of elicited neurotransmitter release. Trends Neurosci. 1997. 20:204–212.
41. Jeon D, Kim C, Yang YM, Rhim H, Yim E, Oh U, Shin HS. Impaired long-term memory and long-term potentiation in N-type Ca2+ channel-deficient mice. Genes Brain Behav. 2007. 6:375–388.
42. Gross RA, Macdonald RL. Dynorphin A selectively reduces a large transient (N-type) calcium current of mouse dorsal root ganglion neurons in cell culture. Proc Natl Acad Sci USA. 1987. 84:5469–5473.
43. Scroggs RS, Fox AP. Multiple Ca2+ currents elicited by action potential waveforms in acutely isolated adult rat dorsal root ganglion neurons. J Neurosci. 1992. 12:1789–1801.
44. Bowersox SS, Gadbois T, Singh T, Pettus M, Wang YX, Luther RR. Selective N-type neuronal voltage-sensitive calcium channel blocker, SNX-111, produces spinal antinociception in rat models of acute, persistent and neuropathic pain. J Pharmacol Exp Ther. 1996. 279:1243–1249.
45. Liu L, Zwingman TA, Fletcher CF. In vivo analysis of voltage-dependent calcium channels. J Bioenerg Biomembr. 2003. 35:671–685.
46. Ino M, Yoshinaga T, Wakamori M, Miyamoto N, Takahashi E, Sonoda J, Kagaya T, Oki T, Nagasu T, Nishizawa Y, Tanaka I, Imoto K, Aizawa S, Koch S, Schwartz A, Niidome T, Sawada K, Mori Y. Functional disorders of the sympathetic nervous system in mice lacking the alpha 1B subunit (Cav 2.2) of N-type calcium channels. Proc Natl Acad Sci USA. 2001. 98:5323–5328.
47. Devor DC, Sekar MC, Frizzell RA, Duffey ME. Taurodeoxycholate activates potassium and chloride conductances via an IP3-mediated release of calcium from intracellular stores in a colonic cell line (T84). J Clin Invest. 1993. 92:2173–2181.
48. Lee HK, Lee KH. Bile acid modulation of gastroinstinal smooth muscle contraction and ionic currents. Korean J Physiol Pharmacol. 2000. 4:333–338.
49. Wehner F. Taurocholate depolarizes rat hepatocytes in primary culture by increasing cell membrane Na+ conductance. Pflugers Arch. 1993. 424:145–151.