Abstract
Graphical Abstract
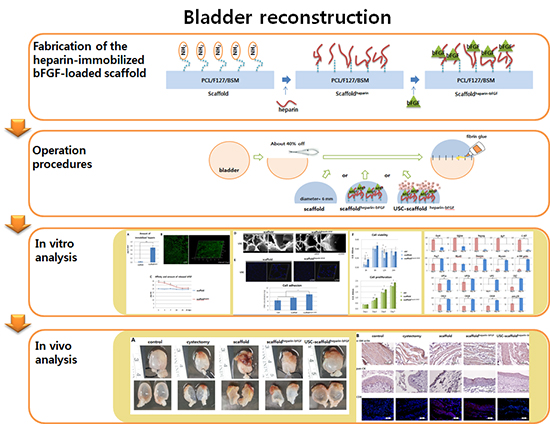
Figures and Tables
Fig. 1
Schematic diagram of the scaffold fabrication and operation procedures. (A) Procedures for fabrication of the heparin-immobilized bFGF-loaded scaffolds (Scaffoldheparin-bFGF) consisting of Polycaprolactone/Pluronic F127/bladder submucosa matrix (PCL/F127/BSM). (B) Bladder reconstruction operation procedure using the various scaffolds.

Fig. 2
Measurement of heparin, bFGF, and scaffoldheparin-bFGF biocompatibility. (A) Amount of immobilized heparin on the scaffold (n=3). (B) Visualization of immobilized heparin on the scaffold. (C) Affinity and amount of bFGF released from the heparin-immobilized scaffold for 28 days. An unmodified heparin scaffold was used as a control. (D) Field emission scanning electron microscope images of scaffold morphology and cells attached to the scaffold. (E) Cell adhesion to the scaffolds was determined by measuring the DNA concentration with Hoechst 33258 staining in confocal images. (F) Biocompatibility analysis of the scaffolds. Ctrl, culture plate dish; scaffold, unmodified scaffold; scaffoldheparin-bFGF, heparin-immobilized bFGF-loaded scaffold; USCs, urine derived stem cells; O.D., optical density. All data are presented as mean±SD (*P<0.01; †P<0.05).

Fig. 3
Cell differentiation supported by the scaffoldheparin-bFGF. (A) USC differentiation (%) into smooth muscle and urothelial cells on the scaffoldheparin-bFGF and representative FACS images. (B) Real-time PCR analysis of stem cell, myogenic, and urothelial lineage markers at days 0 and 14. *P<0.01.
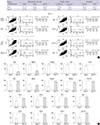
Fig. 4
Morphological and immunohistochemical (IHC) analysis. (A) Morphological analysis of retrieved bladder. (B) Analysis of α-SM actin, pan-CK, CD8, and HuNu expression with IHC. α-SM actin, α-smooth muscle actin; pan-CK, pan-cytokeratin; CD8, cluster of differentiation 8; HuNu, Human nuclei-specific antibody. Control, sham operated; partial cystectomy, approximately 40% defect was created in the dome of the bladder wall; scaffold, unmodified scaffold was attached after partial cystectomy; scaffoldheparin-bFGF, the heparin-immobilized bFGF-loaded scaffold was attached after partial cystectomy; USC-scaffoldheparin-bFGF, scaffoldheparin-bFGF combined with USCs was attached after partial cystectomy. Scale bar=50 µm. Magnification, 400 ×.

Table 1
Primer sequences

Table 2
Measurement of cross-sectional area and urodynamic results, including maximal bladder capacity and compliance

Notes
Funding This research was supported by Basic Science Research Program through the National Research Foundation of Korea (NRF) funded by the Ministry of Science, ICT & Future Planning (grant number) (2014R1A1A3049460); (NRF-2014M3A9D3033887); funded by the Ministry of Education (2015R1D1A3A03020378); and supported by a grant of the Korea Health Technology R&D Project through the Korea Health Industry Development Institute (KHIDI), funded by the Ministry of Health & Welfare (HI14C1642)
AUTHOR CONTRIBUTION Conceived and designed the experiments: Lee JN, Chun SY. Performed the experiments: Lee HJ, Jang YJ, Kim DH. Scaffold manufacturing: Oh SH, Lee JH. Analyzed the data: Song PH. Drafting of the manuscript: Chun SY, Lee JN. Critical revision of the manuscript for important intellectual content: Kwon TG. Statistical analysis: Lee JN. Obtaining funding: Kwon TG. Administrative, technical, or material support: Song PH, Kim DH. Approval of the final manuscript: Kwon TG.