Abstract
Figures and Tables
Fig. 1
Overall procedures used in the conventional intensity-modulated radiation therapy (IMRTconv) and an apparent diffusion coefficient (ADC) map-based IMRT (IMRTADC) plans. The left flow chart connected with black lines describes the general and common procedures to create two IMRT plans. The blue dashed line corresponds to the IMRTconv plan. Additionally required procedures for the IMRTADC were presented with orange line.
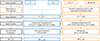
Fig. 2
The delineated target volumes in the intensity-modulated radiation therapy plan. The ADC-based high-risk clinical target volume (aCTVHR), contrast enhanced T1 image-based gross tumor volume (tGTV) and CTV (tCTV). The aCTVHR is defined on ADC maps by applying the ADC criteria for high-grade glioma to extract the high-risk residual target volume. The tCTV is defined by adding a 2-cm margin to the tGTV.
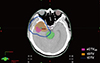
Fig. 3
Multi-modal and post-processed images used to determine the high-risk tumor volume in a high-grade glioma. (A) Computed tomography image. (B) Contrast enhanced-T1 weighted image. (C) Diffusion-weighted (DW) image (b=1,000 s/mm2). (D) ADC map. (E) DW ratio map with normalized average diffusion values of the contralateral normal brain tissues. The red and orange regions represent double- and triple-restricted water diffusion, respectively. (F) Extracted malignant residual tumor volumes on ADC maps with quantitative analysis for suspicious high-risk lesions.
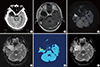
Fig. 4
Comparison of the dose distributions in the IMRTconv plan and IMRTADC plan. (A) Dose distribution in the IMRTADC plan. Prescribed doses of 59.4 Gy and 50.4 Gy were optimized to the aCTVHR and relative complement volume of aCTVHR in tCTV (sCTV), respectively, using the simultaneous integrated boost technique. (B) Dose distribution in the IMRTconv plan. A dose of 59.4 Gy was prescribed to the tCTV.
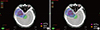
Fig. 5
Comparison of the dose volume histograms (DVHs) in the IMRTconv and the IMRTADC plans. (A) Differential DVHs for the residual clinical target volumes at high risk on the ADC maps. Horizontal axis: doses normalized to the prescribed dose (59.4 Gy). (B) Cumulative DVHs for organs at risk.
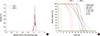
Table 1
Planning parameters for conventional intensity-modulated radiation therapy (IMRTconv) and IMRT based on apparent diffusion coefficient (ADC) maps (IMRTADC). Both plans were based on contrast enhanced (CE)-T1 weighted images

*CE-T1, contrast enhanced-T1 weighted; †DW-MR, diffusion weighted-magnetic resonance; ‡aCTVHR, clinical target volume (CTV) at high risk determined based on the ADC maps; §ADC, apparent diffusion coefficient; ∥CT, computed tomography; ¶sCTV, the relative complement volume of aCTVHR in tCTV, tCTV- (tCTV∩aCTVHR); **SIB, simultaneous integrated boost; ††tCTV, CTV delineated on the CE-T1 images by expanding gross tumor volume with a 2-cm margin.
Table 2
Evaluation of dose distributions using homogeneity (s-index) and conformity indices (conformity number) for the target volumes, aCTVHR showing malignancy of high-grade gliomas on ADC maps and tCTV defined on CE-T1 images
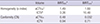
Volume | IMRTADC* | IMRTconv† | |
---|---|---|---|
Homogeneity (s-index) | aCTVHR‡ | 1.49 | 1.60 |
tCTV§ | 3.26 | 10.48 | |
Conformity (CN) | aCTVHR | 0.48 | 0.032 |
tCTV | 0.94 | 0.71 |
*IMRTADC, intensity-modulated radiation therapy (IMRT) plan optimized to aCTVHR and tCTV using simultaneous integrated boost; †IMRTconv, conventional IMRT plan using CE-T1 images for tCTV; ‡aCTVHR, clinical target volume at high risk defined on the ADC maps; §tCTV, expanded tGTV (gross tumor volume on CE-T1 images) with a 2-cm margin.
Table 3
Evaluation of the equivalent uniform doses (EUDs) for organs at risk (OARs) and EUD-based figure-of-merit (f-EUD) in the IMRTADC and the IMRTconv plans
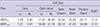
Plan | EUD [Gy] | |||||||
---|---|---|---|---|---|---|---|---|
Lens | Optic nerve | Optic Chiasm | Brain Stem | Pituitary Gland | fEUD* | |||
Lt. | Rt. | Lt. | Rt. | |||||
IMRTADC | 1.09 | 3.40 | 17.87 | 36.09 | 35.56 | 35.95 | 30.17 | 0.14 |
IMRTconv | 1.21 | 3.91 | 18.60 | 41.19 | 39.12 | 41.62 | 34.99 | 0.12 |
Notes
Funding This research was supported by the Leading Foreign Research Institute Recruitment Program through the National Research Foundation of Korea (NRF) funded by the Ministry of Science, ICT & Future Planning (MSIP) (Grant No. 2009-00420).
AUTHOR CONTRIBUTION Conception and design of the study: Lee JW, Ahn KJ, Choe BY, Park JY. Coordination of the study: Hong S, Suh TS, Choe BY. Case selection, image acquisition and interpretation: Ahn KJ. Radiation treatment planning and analysis: Park JY, Lee JW, Park HJ, Hong S. Manuscript preparation: Park JY, Lee JW. Manuscript revision and editing: Suh TS, Lee JW, Ahn KJ, Choe BY, Hong S, Park HJ. Manuscript approval: all authors.
Appendices
APPENDIX
APPENDIX A
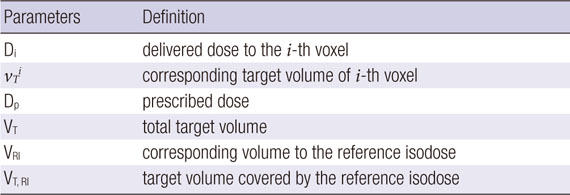
APPENDIX B
Radiobiological parameters in the following table are used to estimate the equivalent uniform dose (EUD) and tumor control probability (TCP) for principal structures.
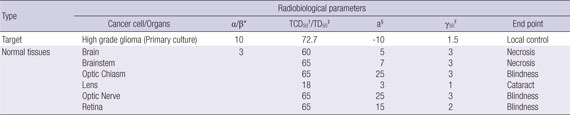