Abstract
The aim of our study was to investigate the differential effects of dexamethasone (DXM) and hydrocortisone (HCS) on somatic growth and postnatal lung development in a rat model of bronchopulmonary dysplasia (BPD). A rat model of BPD was induced by administering intra-amniotic lipopolysaccharide (LPS) and postnatal hyperoxia. The rats were treated with a 6-day (D1-D6) tapering course of DXM (starting dose 0.5 mg/kg/day), HCS (starting dose 2 mg/kg/day), or an equivalent volume of normal saline. DXM treatment in a rat model of BPD induced by LPS and hyperoxia was also associated with a more profound weight loss compared to control and LPS + O2 groups not exposed to corticosteroid, whereas HCS treatment affected body weight only slightly. Examination of lung morphology showed worse mean cord length in both LPS + O2 + DXM and LPS + O2 + HCS groups as compared to the LPS + O2 alone group, and the LPS + O2 + DXM group had thicker alveolar walls than the LPS + O2 group at day 14. The HCS treatment was not significantly associated with aberrant alveolar wall thickening and retarded somatic growth. The use of postnatal DXM or HCS in a rat model of BPD induced by intra-amniotic LPS and postnatal hyperoxia appeared detrimental to lung growth, but there was less effect in the case of HCS. These findings suggest that effect of HCS on somatic growth and pulmonary outcome may be better tolerated in neonates for preventing and/or treating BPD.
Postnatal systemic corticosteroid therapy has been widely used to prevent or decrease the severity of bronchopulmonary dysplasia (BPD) in preterm infants (1, 2), but adverse effects on neurodevelopmental outcomes and lung structure have limited its routine use. Although the use of postnatal systemic corticosteroid therapy for preterm infants at risk of BPD is highly controversial, clinicians continue to use it because it works. Meta-analyses have identified short-term pulmonary benefits of postnatal systemic corticosteroids (3, 4). However work is needed to refine postnatal systemic corticosteroid regimens for preterm infants at risk of developing BPD, in order to enhance pulmonary and neurodevelopmental outcomes while reducing their adverse effects on neurodevelopmental outcome and lung growth. In an attempt to minimize adverse effects, several centers have reduced the cumulative dexamethasone (DXM) dose in infants at greatest risk of BPD.
Recently, it has been suggested that corticosteroids other than DXM, such as hydrocortisone (HCS), might provide comparable benefits with less toxicity (5). Watterberg et al. (6) reported that HCS treatment in preterm infants at corrected age 18-22 month was not associated with growth impairment. Furthermore, low-dose HCS treatment seemed to decrease the incidence of BPD in a pilot trial (7). However, despite the use of lower doses of DXM and adoption of the gentler choice of HCS, serious concerns remain as to their effects on alveolar growth and neurodevelopment (8). This study was designed to evaluate the differential effects of DXM and HCS on somatic growth and alveolar development in a rat model of BPD induced by intra-amniotic lipopolysaccharide (LPS) and postnatal hyperoxia exposure.
The animal experiments were performed at the Clinical Research Institute in Seoul National University Bundang Hospital, Korea, and the protocol was approved by the institutional animal care and use committee (approval number: BA0912-053/048-01). Timed pregnancy Sprague-Dawley rats (term, 22.5 day) weighing 300-360 g were used. The rats were housed in individual cages in the animal unit, and were provided with food and water. Lighting was provided from 6 am to 6 pm. The male:female ratio in the study groups ranged from 0.8 to 1.2, but the differences were not statistically significant. We used a rat model of BPD induced by intra-amniotic LPS and postnatal hyperoxia (9). Pups were divided into 4 groups according to whether or not they received systemic DXM or HCS, as shown in Fig. 1. The study groups were as follows: Control group, no LPS administration and 2 weeks in normal air (n = 18); LPS + O2 group, Intra-amniotic LPS (0.75 µg) administration and 1 week of exposure to 85% oxygen (n = 18); LPS + O2 + DXM group, intra-amniotic LPS (0.75 µg) administration and 1 week of exposure to 85% oxygen plus DXM for 6 day (n = 14); LPS + O2 + HCS group, Intra-amniotic LPS (0.75 µg) administration and 1 week of exposure to 85% oxygen plus HCS for 6 day (n = 12).
On gestation day 20, pregnant rats were anesthetized by isoflurane inhalation. After a midline abdominal incision, 0.75 µg LPS (Escherichia coli 0111:B4; Chemicon International, Temecula, CA, USA) solubilized in 0.05 mL normal saline was injected into the amniotic sacs of the pregnant rats with direct visualization in the LPS + O2, LPS + O2 + DXM, and LPS + O2 + HCS groups. The same volume of normal saline without LPS was injected into the amniotic sacs of pregnant rats in the control group. Rat pups were delivered spontaneously, 24-48 hr after these injections. They were weighed and given to foster rats, 10-12 hr after birth; 5-8 pups were given to each foster rats and the foster rats only reared pups allocated to a single group. The number of pups given to foster mothers was controled to equalize litter size.
In the LPS + O2, LPS + O2 + DXM, and LPS + O2 + HCS groups, the rat pups were placed in cages within 40 L Plexiglas chambers containing 85% oxygen, for 1 week. Oxygen levels were monitored daily using an oxygen sensor (Extech 407510; Extech Instruments Corp., Waltham, MA, USA). After 1 week of hyperoxia, the pups were kept in room air for a second week. The foster rats were switched every 24 hr between the hyperoxic and normoxic chambers to prevent damage to their lungs and provide equal nutrition to each litter. The body weights of the pups were measured on days 1, 7, and 14 after birth.
The day of birth was designated as postnatal day 1. Dexamethasone sodium phosphate (Yuhan Corp., Seoul, Korea) or hydrocortisone succinate (Yuhan Corp.) solubilized in 0.05 mL normal saline was injected intraperitoneally once daily. The DXM-treated rats received a 6-day (D1-D6) tapered course of DMX (0.5 mg/kg/day for 2 day, followed by 0.25 mg/kg/day for 2 day, and 0.125 mg/kg/day for 2 day). The HCS-treated rats also received a 6-day (D1-D6) tapered course (2 mg/kg/day for 2 day, followed by 1 mg/kg/day for 2 day, and 0.5 mg/kg/day for 2 day). Rat pups in the control and LPS + O2 groups received a similar volume of normal saline.
Rat pups were anesthetized by an intraperitoneal injection of ketamine (50 mg/kg; Yuhan Corp) and xylazine (50 mg/kg; Bayer AG, Leverkusen, Germany) on day 14. Their lungs were exposed by thoracotomy and, after exsanguination by transecting the aortas and inferior vena cavas, the right ventricles were punctured and the lungs perfused with 3 mL of phosphate-buffered saline at 25 cm H2O. After removing the lungs, the right lower bronchi were ligated, and the right lower lobes were resected and stored at -80℃ for biochemical analysis. For histological analysis, the trachea were cannulated after removal of the lungs, and buffered formaldehyde (4% paraformaldehyde solubilized in phosphate-buffered saline, pH 7.4) was instilled at 25 cmH2O for 5 min. The tracheas were then closed with sutures and the lungs fixed in buffered formaldehyde for 24 hr at 4℃. Paraffin sections (4 µm) were cut from the right upper and left lobes and mounted onto Super Frost Plus slides (VWR Scientific, West Chester, PA, USA). Slides were then deparaffinized and stained with hematoxylin and eosin (H&E).
Six random non-overlapping fields per pup in two distal lung sections were used for the morphometric examinations. Sections were photographed using a digital camera (Axioskop MRc5; Carl Zeiss, Oberkochen, Germany) attached to an Axioskop 40 microscope (Carl Zeiss) at × 100 magnification and saved as JPEG files. The photographs were analyzed by the morphometric methods designed by Weibel (10). All measurements were made by a single observer unaware of group identities. Tissue volume density (VDT) was determined using a 10 × 10 grid (grid element side length ~29 µm). Mean cord length (Lm) is an estimate of the distance from one airspace wall to another, and was determined by counting intersections of airspace walls, including alveoli, alveolar sacs, and alveolar ducts, with an array of 84 lines, each ~24 µm long. Lung volume was determined by measuring the displacement of water by the lungs after fixation. Alveolar wall thickness (WT) was calculated from WT = VDT × Lm. No corrections were made for perfusion or tissue shrinkage.
Pups were anesthetized by an intraperitoneal injection of ketamine (50 mg/kg, i.p., Yuhan Corp) and xylazine (50 mg/kg, i.p., Bayer AG). The trachea was exposed through a midline incision and, without opening the thorax. BAL fluid was collected by instilling and retrieving a 0.3-mL aliquot of normal saline containing 1 mM EDTA slowly three times on day 14. Total cells were stained with trypan blue and counted. The rat pups from which BAL fluid was obtained were not used for histological analysis in case the BAL procedure had altered the morphology of the lung tissue.
For comparison of survival curves, we performed Kaplan-Meier analyses followed by a log-rank tests. Postnatal changes in body weight, mean cord length, and alveolar wall thickness were expressed as means ± SD. Differences between groups were analyzed by one-way ANOVA followed by post hoc analysis. P values < 0.05 were considered statistically significant.
The overall survival rates of the LPS + O2 and LPS + O2 + HCS groups were not significantly different from that of the control group (74% and 72% vs 90%, P = 0.23 and P = 0.11, respectively). However, the overall survival rate of the LPS + O2 + DXM group was lower than that of the control group (64% vs 90%, P = 0.03). Fig. 2 shows survival data for the four groups, and Fig. 3 displays the body weight gains of the surviving rats on D1, D7, and D14. No significant difference between group body weights was observed immediately after birth, and the mean body weights on D7 and D14 of the LPS + O2 group were not different from those of the control group (D7, 16.8 ± 2.7 g vs 17.2 ± 2.9 g, P = 0.72; D14, 32.7 ± 5.6 g vs 36.0 ± 4.3 g, P = 0.12). The mean body weight on D14 of the LPS + O2 + HCS group was lower than that of the control group, but not different from that of the LPS + O2 group (29.0 ± 4.9 g vs 36.0 ± 4.3 g, P = 0.03; 29.0 ± 4.9 g vs 32.7 ± 5.6 g, P = 0.21). However, the mean body weight of the LPS + O2 + DXM group was significantly lower than those of the control and LPS + O2 groups (D7, 11.9 ± 1.7 g vs 17.2 ± 2.9 g and 16.8 ± 2.7 g, P = 0.03 and P = 0.02; D14, 27.0 ± 4.1 g vs 36.0 ± 4.3 g and 32.7 ± 5.6 g, P = 0.02 and P = 0.03, respectively).
Rats in the control group showed normal alveolarization. However the rats in the LPS + O2, LPS + O2 + DXM, and LPS + O2 + HCS groups, all of which were exposed to hyperoxia, showed reduced alveolarization. Marked inhibition of alveolarization characterized by fewer and larger alveoli was observed in the LPS + O2 + DXM and LPS + O2 + HCS groups compared to the LPS + O2 group. Inflammatory changes, such as inflammatory cell infiltration, intra-alveolar edema, and alveolar destruction, were not observed in any of the experimental groups (Fig. 4).
Lm values, which indicate average alveolar size, were elevated in the LPS + O2, LPS + O2 + DXM, and LPS + O2 + HCS groups compared to the control group (51.2 ± 8.4 µm, 55.9 ± 4.9 µm, and 59.9 ± 7.1 µm vs 45.9 ± 5.3 µm, P = 0.02, P = 0.02, and P = 0.01, respectively), and the LPS + O2 + DXM and LPS + O2 + HCS groups had significantly larger Lm values, than the LPS + O2 group (55.9 ± 4.9 µm and 59.9 ± 7.1 µm vs 51.2 ± 8.4 µm, P = 0.03 and P = 0.02, respectively). There was no significant difference in Lm between the LPS + O2 + DXM and LPS + O2 + HCS groups (Fig. 5A). WT values, defined by the product of tissue volume density and Lm, were greater in the LPS + O2, LPS + O2 + DXM, and LPS + O2 + HCS groups than the control group (16.6 ± 3.4 µm, 21.4 ± 3.6 µm and 18.4 ± 2.7 µm vs 14.3 ± 2.2 µm, P = 0.03, P = 0.01, and P = 0.03, respectively), and the LPS + O2 + DXM group had larger WT than the LPS + O2 and LPS + O2 + HCS groups (21.4 ± 3.6 µm vs 16.6 ± 3.4 µm and 18.4 ± 2.7 µm, P = 0.01 and P = 0.02, respectively). There was no significant difference in WT between the LPS + O2 and LPS + O2 + HCS groups (Fig. 5B).
Cell counts in BAL fluid were significantly higher in the LPS + O2 group than in the control group both on D7 and D14 (D7, × 105 cells/mL vs 47 × 105 cells/mL, P < 0.001; D14, 6 × 105 cells/mL vs 51 × 105 cells/mL, P < 0.001). On D7, the cell counts in BAL fluid in the LPS + O2 + DXM and LPS + O2 + HCS groups were somewhat lower than in the LPS + O2 group, but the differences were not statistically significant. On D14, the LPS + O2 + DXM and LPS + O2 + HCS groups had lower cell counts than the LPS + O2 group (20 × 105 cells/mL and 34 × 105 cells/mL vs 51 × 105 cells/mL, P = 0.02 and P = 0.04, respectively) (Fig. 6).
In this study, DXM treatment in a rat model of BPD induced by LPS and hyperoxia was also associated with a more profound weight loss compared to control and LPS + O2 groups not exposed to corticosteroid, whereas HCS affected body weight only slightly. Examination of lung morphology showed worse mean cord length in both corticosteroid-treated groups as compared to the LPS + O2 group, and especially the DXM-treated group had aberrant alveolar walls thickness than the LPS + O2 group. Total cell count in the BAL was decreased with corticosteroid treatment.
Early systemic treatment with DXM appears to be effective at reducing the risk of BPD in ventilated very low-birth-weight infants (3, 4). Proposed explanations for this improvement include stimulation of surfactant synthesis, increased antioxidant production, decreased lung water, and suppression of lung inflammation (11, 12). However, there is increasing evidence that administration of corticosteroids in preterm infants affects lung development adversely (13-15). Tschanz et al. (16, 17) observed that DXM treatment of rat lungs reduced the interstitial tissue and suppressed the outgrowth of new inter-alveolar septa. Sahebjami and Domino (18) also found that glucocorticoid treatment during the critical period (postnatal D4 to D14) reduced lung growth and development in the long term. Similarly, we found that administration of DXM resulted in fewer and larger airspaces and impaired alveolarization in our rat model of BPD. Taken together, these observations show that neonatal glucocorticoid therapy in the early postnatal period is potentially toxic with respect to lung growth not only of control neonates but also of BPD neonates. Therefore, the pros and cons of glucocorticoid therapy should be carefully weighed before its use in preterm infants at greatest risk of BPD.
In the present study, the significant increase of alveolar wall thickness in DXM-treated group as compared to LPS + O2 group raises the possibility that the glucocorticoids induce abnormal remodeling with increased elastin and collagen in the connective matrix of the alveolar walls. Increased alveolar wall thickness is known to reduce the efficiency of gas exchange. Whether or not the thick connective tissue represents a disordered, nonfunctional regional response needs to be determined. These findings were not in agreement with those reported in rats with no other risk factors for altered lung growth. Thus, Fayon et al. (19) found that in rat pups with no risk factors for BPD, both HCS and DXM increased alveolar diameter, and decreased the thickness of the inter-airspace walls. This thinning of the inter-airspace walls due to precocious maturation prior to alveolarization was more marked with DXM than HCS. Since HCS gave no influence to alveolar septation in the study, they suggested that the lowest effective dose of HCS might replace DXM in premature infants. HCS is the natural hormone, which may be safer to use than the dexamethasone, but randomized trials of lung responses or complications are not available. Watterberg et al. (20) showed that low-dose HCS for 12 day, begun before 48 hr of life in extremely low-birth-weight infants, decreased BPD in a pilot trial. However, the multicenter trial of HCS was discontinued because of an increased incidence of gastrointestinal perforation in the extremely low-birth-weight infants in the HCS group. Additional trials are warranted to identify the lowest effective dose of postnatal HCS and the optimal period of its administration without complication (6, 21).
In our preliminary study, DXM or HCS alone did not affect the significant reduction in somatic growth compared to control group (data not shown). However, postnatal DXM treatment in a rat model of antenatal LPS and neonatal hyperoxia exposure decreased somatic growth of the rat pups in the present study, whereas HCS did not influence body weight. It is well known that decrease in somatic growth is associated with decreased alveolarization (as with starvation) (22). In the murine model, Ohtsu et al. (23) reported that higher doses of DXM (1 and 5 mg/kg/day) are required to affect survival and protect lung from hyperoxia in neonatal rats. We adopted an initial DXM dose of 0.5 mg/kg/day, because it was common initial dose used in the neonatal rat under hyperoxic conditions (24, 25). In published studies (7, 26), the postnatal HCS therapy in preterm infants, administered to prevent or treat BPD, consisted of a starting dose of 1-5 mg/kg/day. In our study, a starting HCS dose of 2 mg/kg/day was relatively low compared to equivalent dose of DXM, but equivalent to dose used in clinical practice. Although data on the pharmacokinetics of HCS in neonatal animals are even more limited than that of DXM, it may still be higher than what is needed to achieve the desired effect without long-term adverse effects. Further extensive studies are needed to determine the lowest effective dose of HCS for lung development before HCS can be considered an alternative to DXM for preventing and/or treating BPD. We believe that our rat pup model of BPD could be used to examine several doses of interventions to determine the effects on alveolarization in the absence of effect on mortality and somatic growth.
In the present study, both DXM and HCS significantly decreased cell counts in BAL fluid on D14 compared to the LPS + O2 group. There is a possibility that total cells in BAL fluid, including alveolar macrophages, polymorphonuclear leukocytes, lymphocytes are correlated with pulmonary inflammation. It is known that bronchial and alveolar epithelial cells, together with inflammatory cells, secrete several proinflammatory cytokines into the BAL fluid of infants who subsequently develop BPD (27). The increased cell count in the BAL fluid may consist mainly of neutrophils, and to a lesser degree, epithelial cells, alveolar macrophages, and lymphocytes (28). Glucocorticoids seem to be effective in regulating inflammatory factors by inhibiting binding of specific transcription factors such as nuclear factor κB and activated protein 1 (11). Indeed, inflammatory cells and levels of chemokines and cytokines in bronchoalveolar fluid decrease after DXM treatment (29).
Yoder et al. (1) reported that pulmonary inflammation was suppressed by DXM in ventilator-dependent preterm infants. They suggested that short-term treatment with DXM improved pulmonary function, suppressed pulmonary inflammation, and reduced the need for respiratory support in ventilator-dependent preterm infants. However, even though DXM or HCS reduced pulmonary inflammation in our study, that did not protect the lungs from their negative effects in terms of alveolar growth. Fayon et al. (19) compared the effect of DXM and HCS on lung growth with a lower DXM dose than we used, and they also found that alveolarization was more markedly impaired by DXM than HCS. Dik et al. (30) demonstrated that DXM treatment increased fibroblast proliferation despite apparent down-regulation of inflammation in preterm infants at risk of BPD. In the present study, there was a difference between DXM and HCS in terms of adverse effect on alveolar development. The use of postnatal DXM or HCS in a rat model of BPD induced by intra-amniotic LPS and postnatal hyperoxia led to interrupted alveolarization, but HCS showed less aberrant thickening of the alveolar wall than DXM.
In summary, unlike the DXM-treated group, the HCS-treated group in a rat model of antenatal LPS and postnatal hyperoxia was not associated with aberrant alveolar wall thickening and retarded somatic growth. The effect of HCS on alveolarization in the absence of effect on somatic growth needs to be better defined under several doses of interventions. The lowest possible effective dose in the shortest time may reduce pulmonary inflammation without inhibiting normal lung growth.
Figures and Tables
Fig. 1
Schematic outline of the experimental protocol. LPS, lipopolysaccharide; L (0.75), lipopolysaccharide administered at 0.75 µg at gestation day 20; O2, exposure to 85% oxygen for 1 week; DXM, dexamethasone administered with a 6-day tapered course; HCS, hydrocortisone administered at a 6-day tapered course.
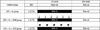
Fig. 2
Postnatal survival rates in the experimental groups. LPS, lipopolysaccharide; O2, exposure to 85% oxygen for 1 week; DXM, dexamethasone; HCS, hydrocortisone. *Significantly higher or lower than the control group.
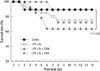
Fig. 3
Postnatal weight gain in the experimental groups. Black, gray, and white bars indicate body weights on days 1, 7, and 14, respectively. Data are based on survival to day 14. LPS, lipopolysaccharide; O2, exposure to 85% oxygen for 1 week; DXM, dexamethasone; HCS, hydrocortisone. *Significantly higher or lower than the control group; †Significantly higher or lower than the LPS + O2 group.
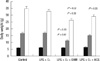
Fig. 4
Representative photomicrographs of rat lungs on day 14. H&E stained. Magnification × 100. Scale bars indicate 200 µm. LPS, lipopolysaccharide; O2, exposure to 85% oxygen for 1 week; DXM, dexamethasone; HCS, hydrocortisone.
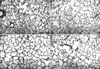
Fig. 5
Morphometric data. Mean cord length (Lm) (A) and alveolar wall thickness (WT) (B). LPS, lipopolysaccharide; O2, exposure to 85% oxygen for 1 week; DXM, dexamethasone; HCS, hydrocortisone. *Significantly higher or lower than the control group; †Significantly higher or lower than the LPS + O2 group; ‡Significantly higher or lower than the LPS + O2 + HCS group.
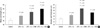
References
1. Yoder MC Jr, Chua R, Tepper R. Effect of dexamethasone on pulmonary inflammation and pulmonary function of ventilator-dependent infants with bronchopulmonary dysplasia. Am Rev Respir Dis. 1991. 143:1044–1048.
2. Halliday HL. Clinical trials of postnatal corticosteroids: inhaled and systemic. Biol Neonate. 1999. 76:29–40.
3. Halliday HL, Ehrenkranz RA, Doyle LW. Early postnatal (< 96 hours) corticosteroids for preventing chronic lung disease in preterm infants. Cochrane Database Syst Rev. 2003. CD001146.
4. Halliday HL, Ehrenkranz RA. Moderately early (7-14 days) postnatal corticosteroids for preventing chronic lung disease in preterm infants. Cochrane Database Syst Rev. 2001. CD001144.
5. Doyle LW, Ehrenkranz RA, Halliday HL. Postnatal hydrocortisone for preventing or treating bronchopulmonary dysplasia in preterm infants: a systematic review. Neonatology. 2010. 98:111–117.
6. Watterberg KL, Shaffer ML, Mishefske MJ, Leach CL, Mammel MC, Couser RJ, Abbasi S, Cole CH, Aucott SW, Thilo EH, Rozycki HJ, Lacy CB. Growth and neurodevelopmental outcomes after early low-dose hydrocortisone treatment in extremely low birth weight infants. Pediatrics. 2007. 120:40–48.
7. Watterberg KL, Gerdes JS, Gifford KL, Lin HM. Prophylaxis against early adrenal insufficiency to prevent chronic lung disease in premature infants. Pediatrics. 1999. 104:1258–1263.
8. Thébaud B, Lacaze-Masmonteil T, Watterberg K. Postnatal glucocorticoids in very preterm infants: "the good, the bad, and the ugly"? Pediatrics. 2001. 107:413–415.
9. Choi CW, Kim BI, Hong JS, Kim EK, Kim HS, Choi JH. Bronchopulmonary dysplasia in a rat model induced by intra-amniotic inflammation and postnatal hyperoxia: morphometric aspects. Pediatr Res. 2009. 65:323–327.
10. Weibel ER. Principles and methods for the morphometric study of the lung and other organs. Lab Invest. 1963. 12:131–155.
11. Bolt RJ, van Weissenbruch MM, Lafeber HN, Delemarre-van de Waal HA. Glucocorticoids and lung development in the fetus and preterm infant. Pediatr Pulmonol. 2001. 32:76–91.
12. Gross I. Regulation of fetal lung maturation. Am J Physiol. 1990. 259:L337–L344.
13. Vyas J, Kotecha S. Effects of antenatal and postnatal corticosteroids on the preterm lung. Arch Dis Child Fetal Neonatal Ed. 1997. 77:F147–F150.
14. Massaro GD, Massaro D. Formation of alveoli in rats: postnatal effect of prenatal dexamethasone. Am J Physiol. 1992. 263:L37–L41.
15. Tschanz SA, Makanya AN, Haenni B, Burri PH. Effects of neonatal high-dose short-term glucocorticoid treatment on the lung: a morphologic and morphometric study in the rat. Pediatr Res. 2003. 53:72–80.
16. Tschanz SA, Burri PH. Postnatal lung development and its impairment by glucocorticoids. Pediatr Pulmonol Suppl. 1997. 16:247–249.
17. Tschanz SA, Damke BM, Burri PH. Influence of postnatally administered glucocorticoids on rat lung growth. Biol Neonate. 1995. 68:229–245.
18. Sahebjami H, Domino M. Effects of postnatal dexamethasone treatment on development of alveoli in adult rats. Exp Lung Res. 1989. 15:961–973.
19. Fayon M, Jouvencel P, Carles D, Choukroun ML, Marthan R. Differential effect of dexamethasone and hydrocortisone on alveolar growth in rat pups. Pediatr Pulmonol. 2002. 33:443–448.
20. Watterberg KL, Gerdes JS, Cole CH, Aucott SW, Thilo EH, Mammel MC, Couser RJ, Garland JS, Rozycki HJ, Leach CL, Backstrom C, Shaffer ML. Prophylaxis of early adrenal insufficiency to prevent bronchopulmonary dysplasia: a multicenter trial. Pediatrics. 2004. 114:1649–1657.
21. Rademaker KJ, Uiterwaal CS, Groenendaal F, Venema MM, van Bel F, Beek FJ, van Haastert IC, Grobbee DE, de Vries LS. Neonatal hydrocortisone treatment: neurodevelopmental outcome and MRI at school age in preterm-born children. J Pediatr. 2007. 150:351–357.
22. Leitch CA, Ahlrichs J, Karn C, Denne SC. Energy expenditure and energy intake during dexamethasone therapy for chronic lung disease. Pediatr Res. 1999. 46:109–113.
23. Ohtsu N, Ariagno RL, Sweeney TE, Davis L, Moses L, Petriceks S, Daehne I, Bensch K, Northway WH Jr. The effect of dexamethasone on chronic pulmonary oxygen toxicity in infant mice. Pediatr Res. 1989. 25:353–359.
24. Dallas DV, Keeney SE, Mathews MJ, Schmalstieg FC. Effects of postnatal dexamethasone on oxygen toxicity in neonatal rats. Biol Neonate. 2004. 86:145–154.
25. Ohashi T, Takada S, Motoike T, Tsuneishi S, Matsuo M, Sano K, Nakamura H. Effect of dexamethasone on pulmonary surfactant metabolism in hyperoxia-treated rat lungs. Pediatr Res. 1991. 29:173–177.
26. Lodygensky GA, Rademaker K, Zimine S, Gex-Fabry M, Lieftink AF, Lazeyras F, Groenendaal F, de Vries LS, Huppi PS. Structural and functional brain development after hydrocortisone treatment for neonatal chronic lung disease. Pediatrics. 2005. 116:1–7.
27. Ozdemir A, Brown MA, Morgan WJ. Markers and mediators of inflammation in neonatal lung disease. Pediatr Pulmonol. 1997. 23:292–306.
28. Deng H, Mason SN, Auten RL Jr. Lung inflammation in hyperoxia can be prevented by antichemokine treatment in newborn rats. Am J Respir Crit Care Med. 2000. 162:2316–2323.
29. Groneck P, Reuss D, Gotze-Speer B, Speer CP. Effects of dexamethasone on chemotactic activity and inflammatory mediators in tracheobronchial aspirates of preterm infants at risk for chronic lung disease. J Pediatr. 1993. 122:938–944.
30. Dik WA, Versnel MA, Naber BA, Janssen DJ, van Kaam AH, Zimmermann LJ. Dexamethasone treatment does not inhibit fibroproliferation in chronic lung disease of prematurity. Eur Respir J. 2003. 21:842–847.