Abstract
The cellular toxicities of surfactants, a solvent, and an antifreeze that are included in herbicide formulations were assessed by measuring their effects on membrane integrity, metabolic activity, mitochondrial activity, and total protein synthesis rate in a cell culture. Polyethylene glycol, propylene glycol, and monoethylene glycol exhibited no cellular toxicity even at a high concentration of 100 mM. Sodium lauryl ether sulfate and polyoxyethylene lauryl ether significantly damaged the membrane, disturbed cellular metabolic activity, and decreased mitochondrial activity and the protein synthesis rate; however, their toxicity was far below those of the severely toxic chemicals at comparable concentrations. The severely toxic category included polyoxypropylene glycol block copolymer, polyoxyethylene tallow amine, and polyoxyethylene lauryl amine ether. These surfactants were cytotoxic between 3.125 µM and 100 µM in a dose-dependent manner. However, the toxicity graph of concentration vs toxicity had a point of inflection at 25 µM. The slope of the toxicity graph was gentle when the concentration was below 25 µM and steep when the concentration was greater than 25 µM. In conclusion, our results suggest that the toxicity of surfactants be taken care of pertinent treatment of acute herbicide intoxication.
Herbicides prevent the growth of noxious or unwanted weeds. Because of their benefits, the use of herbicides has grown continuously over the last several decades internationally. As farmers have been able to more easily control vegetation, their crops have grown in greater quantities. However, the negative effects of herbicide use pose serious social issues in Asian countries (1-4). Farmers sometimes accidentally or willfully swallow toxic concentrations of herbicides, which results in death or severe detrimental effects.
The ability of herbicides to control certain kinds of weeds depends on the chemical and biological properties of the agent used. Fortunately, because of the differences in the pathways of chemical reactions and/or metabolism between plants and humans, some herbicides that are highly toxic to plants are only minimally toxic to mammals with a few exceptions including paraquat. However, clinical toxicologists sometimes encounter unpredictable cases of severe toxicity. Patients who have ingested reportedly low levels of toxic herbicides sometimes become severely ill. For example, although glyphosate and glufosinate ingredients are known to be minimally toxic to humans, ingestion of large amounts of these products have caused patients to die or to suffer from the effects of severe toxicity such as respiratory distress, metabolic acidosis, tachycardia, renal failure, and electrolyte imbalances (5). In these cases, surfactants are believed to be the chemicals responsible for such adverse effects (6-15).
Surfactants are a large group of surface-active substances that have a wide variety of applications. They each have a hydrophobic and hydrophilic component. The hydrophobic part consists of an uncharged carbohydrate group that can be straight, branched, cyclic, or aromatic (16). Surfactants are classified as nonionic, anionic, cationic, or amphoteric depending on the nature of their hydrophilic component.
During the last 2 decades, extensive research has been carried out to investigate the nature of "glyphosate-surfactant toxicity" in clinical toxicology (3, 17, 18). Several possible mechanisms for this toxicity have been proposed from the findings of these studies because the surfactants might cause direct cellular injury or alter the toxicity of the herbicide. However, the end products of herbicides are comprised of different chemicals for various purposes; these are summarized in Table 1. Therefore, it remains unclear whether the surfactants themselves are entirely responsible for so-called "surfactant toxicity." This issue will not be resolved until we have toxicological information on the other chemicals used in herbicide formulation besides surfactants.
Herbicide formulations differ by manufacturer and nation. Manufacturers are sometimes reluctant to provide the precise formulations they use for their herbicides, including the type and concentration of surfactants used. Due to the lack of precise toxicological information on the chemicals used in herbicides and specific treatment guidelines, clinicians cannot reliably treat patients with suspected "acute herbicide-surfactant intoxication."
Recently, we identified 6 surfactants, 1 solvent, and 1 antifreeze (Table 2) that are commonly included in the formulation of herbicides in Korea. The present study was designed to clarify the cellular toxicities of these surfactants and compare them with those of the solvent and antifreeze.
Six surfactants (1 anionic and 5 nonionic), 1 solvent, and 1 antifreeze, used as an adjuvant for surfactants in herbicide formulation were investigated in this study (Table 2). All of these chemicals were obtained from the major suppliers of chemicals to a manufacturer of herbicides in Korea (Coseal Co., Goonsan, Korea). More specifically, Honam Petrochemical Co., (Seoul, Korea) provided polyethylene glycol (PEG 300) and monoethylene glycol (MEG); SKC (Seoul, Korea) provided propylene glycol (PG); and KPX Green Chemical Co., (Seoul, Korea) provided polyoxyethylene lauryl amine ether (LN-10), sodium lauryl ether sulfate (SLES), poly(oxyethylene, oxypropylene)glycol block copolymer (PE-61), polyoxyethylene lauryl ether (LE-2), and polyoxyethylene tallow amine (TN-20).
Mouse fibroblast-like cells, L-929 (ATCC, CCL-1), derived from mouse fibroblasts were used to assess cytotoxicity and cell viability. L-929 cells were maintained and cultured in RPMI medium (RPMI-1640; HyClone, Logan, UT, USA) containing 10% heat-inactivated fetal bovine serum (FBS; Gibco, Rockville, MD, USA), 2 mM L-glutamine (Sigma, St. Louis, MO, USA), and 100 U/mL penicillin (Sigma, St. Louis, MO, USA) in an incubator containing 5% CO2 at 37℃. EDTA-trypsin (Sigma, St. Louis, MO, USA) was used to detach the cells from a T-75 flask. The cells were counted under a light microscope using Trypan blue stain and a hemocytometer.
The range of each chemical concentration for the cytotoxicity test was derived from the inhibitory concentration 50 (IC50) with respect to relative cell viability (%). The cellular viability (%) of the surfactants, solvent, and antifreeze, which are summarized in Table 2, was determined using the MTT [3-(4,5-dimethylthiazol-2-yl)-2,5-diphenyltetrazolium bromide] assay following the standard testing protocol outlined in the International Organization for Standardization (ISO) 10993-5 guidelines. In brief, cells (1 × 104 cells/mL) were exposed to various concentrations of test samples in a 24-well plate previously sub-cultured in a CO2 incubator containing 5% CO2 at 37℃ for 72 hr. After 72 hr of incubation, 100 µL 5 mg/mL MTT solution (Sigma, St. Louis, MO, USA) was added to the wells and re-incubated for 4 hr. The supernatant was then removed, and 1 mL dimethyl sulfoxide (DMSO) was added to each well. As soon as purple formazan crystals formed and dissolved, the solution was collected and pipetted into a 96-well plate. The absorbance was subsequently measured at 595 nm using an ELISA reader. Cell viability was measured as the ratio of the optical density in the medium containing the extract solution to that in fresh medium.
Cytotoxicity and cell viability tests were performed using the In Cytotox-LDH-GLU-XTT-SRB 4-Parameter Cytotoxicity Kit (Xenometrix AG, Gewerbertrasse 25, Switzerland), which measures membrane integrity (extracellular lactate dehydrogenase [LDHe]), metabolic activity (glucose [GLU]), mitochondrial activity (tetrazolium hydroxide [XTT]), and total protein synthesis rate (sulforhodamine B [SRB]) in a single-cell-type culture.
Previously sub-cultured L-929 cells (1 × 104 cells/mL) were exposed to 5 surfactants at concentrations ranging from 3.125 M to 100 nM, and 1 surfactant, solvent, and antifreeze each at concentrations ranging from 6.25 to 100 mM (Table 2). The cells were incubated with the test samples in a 5% CO2 incubator at 37℃ for 72 hr.
Lactate dehydrogenase (LDH) is a stable cytoplasmic enzyme present in all cells and is rapidly released into the cell culture supernatant upon membrane damage or cell lysis. LDH reduces pyruvate to lactate by oxidizing NADH to NAD+. The consumption of NADH can be measured by spectrophotometry. The results are expressed as the relative toxicity compared to that of 1% Triton X-100 (control).
Cultured cells continuously consume glucose from their culture media for the generation of biosynthetic intermediates. Thus, extracellular glucose concentration is inversely proportional to the rate of glucose uptake, which is an indicator of the functional metabolic state of cells.
XTT [2,3-bis(-methoxy-4-nitro-5-sulfophenyl)-2H-tetrazolium-5-carboxyanilide inner salt] is a tetrazolium salt that is cleaved to formazan by the succinate dehydrogenase system, which belongs to the mitochondrial respiratory chain and is only active in viable cells. Mitochondrial succinate dehydrogenase reduces the yellow tetrazolium salt into soluble orange formazan in the presence of an electron-coupling reagent.
Cell proliferation, which was measured as total protein synthesis, is a very sensitive toxicological marker. Sulforhodamine B (SRB) is an anionic dye that binds electrostatically to cellular proteins. Fixed SRB dye, measured photometrically after solubilization, correlates to the total protein synthesis rate and, therefore, cell proliferation.
Each experiment was repeated 4 times. The data are presented as mean ± standard error. Data between groups were compared using the Kruskal-Wallis test, and P < 0.05 was considered significant. IC50 values were estimated by non-linear regression analysis using Prism (GraphPad Software, San Diego, CA, USA).
The IC50 values for MEG, PEG 300, SLES, PG, LE-2, TN-20, LN-10, and PE-61 were 1.527 mM, 0.70 mM, 602 µM, 394.7 µM, 322 µM, 78.2 µM, 63.2 µM, and 49 µM, respectively. Using these values, the treatment conentrations to assess the toxicity of these chemicals to cellular organelles ranged from 3.125 µM to 100 mM (Fig. 1).
Membrane damage, which was assessed by LDH, was not observed with PEG, PG, or MEG, while the 5 other surfactants were toxic to the cell membrane from 6.25 to 100 µM in a concentration-dependent manner. The membrane toxicities (with 1% Triton X-100 as reference) of the 5 surfactants were as follows: at 25 µM, 8.8% ± 0.6% for LN-10, 0.3% ± 0.1% for SLES, 0.7% ± 0.2% for PE-61, 6.3% ± 0.1% for LE-2, and 8.0% ± 0.5% for TN-20 and at 50 µM, 27.7% ± 1.7% for LN-10, 6.2% ± 0.7% for SLES, 28.7% ± 0.6% for PE-61, 6.5% ± 0.4% for LE-2, and 104.5% ± 7.5% for TN-20. TN-20, LN-10, and PE-61 were highly toxic to the cell membrane at concentrations exceeding 25 µM. Among these chemicals, TN-20 was the most toxic surfactant to the cell membrane (P < 0.01) (Fig. 2, 3).
The cellular metabolic activity, which was assessed by glucose level, was not disrupted by PEG, PG, or MEG. However, the other 5 surfactants suppressed metabolic activity at concentrations between 6.25 and 100 µM in a concentration-dependent manner. The effect of each surfactant on metabolic activity was as follows: at 25 µM, 81.8% ± 7.1% for LN-10, 91.0% ± 7.1% for SLES, 75.7% ± 7.2% for PE-61, 91.2% ± 7.7% for LE-2, and 98.0% ± 5.5% for TN-20; and at 50 µM, 60.9% ± 8.6% for LN-10, 90.2% ± 2.1% for SLES, 25.4% ± 4.6% for PE-61, 88.5% ± 7.1% for LE-2, and 132.5% ± 2.5% for TN-20. In particular, PE-61, TN-20, and LN-10 significantly suppressed cellular metabolic activity at concentrations above 25 µM (P < 0.05) (Fig. 2, 3).
Mitochondrial activity was not affected by PEG, PG, or MEG. However, the other 5 surfactants suppressed mitochondrial activity at concentrations between 6.25 and 100 µM in a concentration-dependent manner. The effect of each surfactant on mitochondrial activity was as follows: at 25 µM, 88.3% ± 3.0% for LN-10, 92.0% ± 9.1% for SLES, 81.0% ± 8.2% for PE-61, 93.1% ± 5.4% for LE-2, and 96.5% ± 5.1% for TN-20; and at 50 µM, 69.7% ± 7.2% for LN-10, 91.1% ± 2.1% for SLES, 40.4% ± 3.7% for PE-61, 90.6% ± 6.1% for LE-2, and 45.5% ± 2.5% for TN-20. In particular, PE-61, TN-20, and LN-10 significantly suppressed cellular metabolic activity at concentrations above 25 µM (P < 0.05) (Fig. 2, 3).
Similarly, cell proliferation, which was measured as total protein synthesis, was also significantly suppressed by the 5 surfactants, but not by PEG, PG, or MEG. The amount of total protein synthesized for each surfactant was as follows: at 25 µM, 92.9% ± 8.60% for LN-10, 96.5% ± 5.8% for SLES, 52.1% ± 3.7% for PE-61, 94.1% ± 5.0% for LE-2, and 85.4% ± 3.6% for TN-20; and at 50 µM, 50.2% ± 4.8% for LN-10, 92.8% ± 8.4% for SLES, 3.9% ± 1.6% for PE-61, 87.6% ± 8.9% for LE-2, and 5.5% ± 0.5% for TN-20. Similar to the trends observed for membrane integrity, metabolic activity, and mitochondrial activity, the total protein synthesis rate was significantly suppressed by PE-61, TN-20, and LN-10 at concentrations above 25 µM (P < 0.01) (Fig. 2, 3).
Several hundred pesticides are produced in Korea; many of them contain additives, including various kinds of solvents and/or surfactants. In the formulation of herbicides, the majority of surfactants are complexes of more than 2 surfactant ingredients. Furthermore, different surfactants may be the chief surfactant ingredient in a particular herbicide; for example, LN-10, MEG and TN-20 are common surfactants in glyphosate herbicides. In the same way, PE-61 is in glyphosate ammonium, LE-2 is in Fosetyl-aluminum, and SLES is a common surfactant in glufosinate herbicide according to manufacturers. In this study, we chose 6 surfactants that are often included in the formulas of pesticides in Korea. Although PG (an antifreeze) and MEG (a solvent) are not surfactants, they are often included in the formulas of pesticides. Therefore, the toxicities of these 2 chemicals were compared with those of the surfactants in this study.
According to our results, the cytotoxicity of the chemicals could be classified in 1 of the 3 following categories (Fig. 2, 3):
The non-toxic chemicals did not result in membrane damage, altered cellular metabolic activity, decreased mitochondrial activity, or altered protein synthesis rate, even at a high concentration of 100 mM (Fig. 2, 3).
The mildly toxic chemicals significantly damaged the membrane, disturbed cellular metabolic activity, and decreased mitochondrial activity and protein synthesis rate at concentrations between 3.125 and 100 µM. However, the intensity of the toxicity was far below that of the severely toxic chemicals at comparable concentrations (Fig. 2, 3).
The severely toxic category included PE-61, TN-20, and LN-10. This category of surfactants was cytotoxic in a dose-dependent manner between 3.125 and 100 µM. However, the toxicity graph (concentration vs toxicity) exhibited an inflection point at 25 µM. The slope of the toxicity graph was gentle at concentrations less than 25 µM and steep at concentrations greater than 25 µM (Fig. 2, 3). At 50 µM, TN-20 was the most toxic substance against the membrane; meanwhile, PE-61 was the most toxic in terms of metabolic activity, mitochondrial activity, and total protein synthesis (Fig. 2, 3).
We believe that the in vitro cellular toxicities of the tested chemicals are relevant to in vivo toxicity of the heart, kidney, and liver, causing the so-called "surfactant syndrome" more or less.
However, there are some limitations of our study that should be noted. In addition to surfactants, solvents, and antifreeze, herbicides include other chemicals such as antifoaming, supplementary, expansion, and viscosity agents, as well as preservatives. Further experiments should be conducted these chemicals. To extend the results of this study to the clinical goal of developing novel treatment modalities for acute herbicide intoxication, further studies on the metabolism, biotransformation, and toxicokinetics of various target chemicals are necessary.
In summary, the surfactants were more toxic than the solvent and antifreeze in our experiment. Three out of 4 nonionic surfactants (PE-61, TN-20, and LN-10) exhibited strong toxicity in a similar dose-dependent manner between concentrations of 3.125 and 100 µM. The toxicity graph derived from the concentrations of PE-61, TN-20, and LN-10 has an inflection point at 25 µM; the slope increases slowly up to 25 µM before decreasing rapidly. TN-20 was the most toxic chemical against the membrane, but PE-61 was the most toxic with respect to metabolic activity, mitochondrial activity, and total protein synthesis.
In conclusion, our results suggest that for the purpose of pertinent treatment of acute herbicide intoxication, not only the toxicity of the main ingredient but also of the additives of herbicides, particularly surfactants, be taken care of.
Figures and Tables
Fig. 1
Overall cell viability in the presence of each chemical between 500 µM and 500 mM. Note the non-toxicity of PEG 300 (an antifreeze), PG, and MEG (solvents); mild toxicity of SLES (an anionic surfactant) and LE-2 (a nonionic surfactant); and severe toxicity of TN-20, LN-10, and PE-61 (nonionic surfactants).
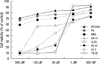
Fig. 2
Effects of the tested compounds on membrane integrity (extracellular lactate dehydrogenase [LDHe]), metabolic activity (glucose [GLU]), mitochondrial activity (tetrazolium hydroxide [XTT]), and total protein synthesis rate (sulforhodamine B [SRB]) at concentrations between 6.25 and 100 µM. Note the non-toxicity of PEG 300 (an antifreeze), PG, and MEG (solvents); mild toxicity of SLES (an anionic surfactant) and LE-2 a nonionic surfactant; and the severe toxicity of TN-20, LN-10, and PE-61 (nonionic surfactants). The data on membrane integrity (LDHe) are presented on the right side of the Y-axis, and range from -20% to 120%, which is the toxicity of 1% Triton-X 100 (control).
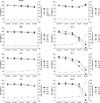
Fig. 3
Comparison of toxicity with respect to membrane integrity (A), metabolic activity (B), mitochondrial activity (C), and total protein synthesis rate (D). Note that PEG 300 (an antifreeze), PG, and MEG (solvents) exhibited no toxicity even at a high concentration of 100 mM. Three out of 4 nonionic surfactants (PE-61, TN-20, and LN-10) exhibited severe toxicity in a similar dose-dependent manner between 3.125 µM and 100 mM. The toxicity graph derived from the concentrations of PE-61, TN-20, and LN-10 has a point of inflection at 25 µM. The slope increases slowly up to 25 µM and decreases rapidly thereafter.
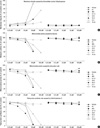
References
1. Seok SJ, Gil HW, Jeong DS, Yang JO, Lee EY, Hong SY. Paraquat intoxication in subjects who attempt suicide: why they chose paraquat. Korean J Intern Med. 2009. 24:247–251.
2. Nesime Y, Lokman B, Akif IM, Gurol C, Basar C, Mustafa K. Acute pesticide poisoning related deaths in Turkey. Vet Hum Toxicol. 2004. 46:342–344.
3. Lee CH, Shih CP, Hsu KH, Hung DZ, Lin CC. The early prognostic factors of glyphosate-surfactant intoxication. Am J Emerg Med. 2008. 26:275–281.
4. Gil HW, Seok SJ, Jeong DS, Yang JO, Lee EY, Hong SY. Plasma level of malondialdehyde in the cases of acute paraquat intoxication. Clin Toxicol (Phila). 2010. 48:149–152.
5. Moon JM, Chun BJ. Predicting acute complicated glyphosate intoxication in the emergency department. Clin Toxicol (Phila). 2010. 48:718–724.
6. Wu JY, Chang SS, Tseng CP, Deng JF, Lee CC. Parenteral glyphosate-surfactant herbicide intoxication. Am J Emerg Med. 2006. 24:504–506.
7. Weng SF, Hung DZ, Hu SY, Tsan YT, Wang LM. Rhabdomyolysis from an intramuscular injection of glyphosate-surfactant herbicide. Clin Toxicol (Phila). 2008. 46:890–891.
8. Temple WA, Smith NA. Glyphosate herbicide poisoning experience in New Zealand. N Z Med J. 1992. 105:173–174.
9. Talbot AR, Shiaw MH, Huang JS, Yang SF, Goo TS, Wang SH, Chen CL, Sanford TR. Acute poisoning with a glyphosate-surfactant herbicide ('Roundup'): a review of 93 cases. Hum Exp Toxicol. 1991. 10:1–8.
10. Palli E, Makris D, Diakaki C, Garoufalis G, Zakynthinos E. Rapture of the large intestine caused by severe oral glyphosate-surfactant intoxication. Am J Emerg Med. 2011. 29:459–460.
11. Menkes DB, Temple WA, Edwards IR. Intentional self-poisoning with glyphosate-containing herbicides. Hum Exp Toxicol. 1991. 10:103–107.
12. Lin CM, Lai CP, Fang TC, Lin CL. Cardiogenic shock in a patient with glyphosate-surfactant poisoning. J Formos Med Assoc. 1999. 98:698–700.
13. Hung DZ, Deng JF, Wu TC. Laryngeal survey in glyphosate intoxication: a pathophysiological investigation. Hum Exp Toxicol. 1997. 16:596–599.
14. Chang CY, Peng YC, Hung DZ, Hu WH, Yang DY, Lin TJ. Clinical impact of upper gastrointestinal tract injuries in glyphosate-surfactant oral intoxication. Hum Exp Toxicol. 1999. 18:475–478.
15. Lee HL, Kan CD, Tsai CL, Liou MJ, Guo HR. Comparative effects of the formulation of glyphosate-surfactant herbicides on hemodynamics in swine. Clin Toxicol (Phila). 2009. 47:651–658.
16. Baeurle SA, Kroener J. Modeling effective interactions of micellar aggregates of ionic surfactants with the Gauss-Core potential. J Math Chem. 2004. 36:409–421.
17. Lee HL, Chen KW, Chi CH, Huang JJ, Tsai LM. Clinical presentations and prognostic factors of a glyphosate-surfactant herbicide intoxication: a review of 131 cases. Acad Emerg Med. 2000. 7:906–910.
18. Bradberry SM, Proudfoot AT, Vale JA. Glyphosate poisoning. Toxicol Rev. 2004. 23:159–167.