Abstract
Neuronal apoptosis induced by amyloid β-peptide (Aβ) plays an important role in the pathophysiology of Alzheimer's disease (AD). However, the molecular mechanism underlying Aβ-induced apoptosis remains undetermined. The disialoganglioside GD3 involves ceramide-, Fas- and TNF-α-mediated apoptosis in lymphoid cells and hepatocytes. Although the implication of GD3 has been suggested, the precise role of GD3 in Aβ-induced apoptosis is still unclear. Here, we investsigated the changes of GD3 metabolism and characterized the distribution and trafficking of GD3 during Aβ-induced apoptosis using human brain-derived TE671 cells. Extracellular Aβ-induced apoptosis in a mitochondrial-dependent manner. GD3 level was negligible in the basal condition. However, in response to extracellular Aβ, both the expression of GD3 synthase mRNA and the intracellular GD3 level were dramatically increased. Neosynthesized GD3 rapidly accumulated in cell surface lipid microdomains, and was then translocated to mitochondria to execute the apoptosis. Disruption of membrane lipid microdomains with methyl-β-cyclodextrin significantly prevented both GD3 accumulation in cell surface and Aβ-induced apoptosis. Our data suggest that rapidly accumulated GD3 in plasma membrane lipid microdomains prior to mitochondrial translocation is one of the key events in Aβ-induced apoptosis.
Alzheimer's disease (AD) is a progressive neurodegenerative disease of the central nervous system associated with deterioration of cognition and memory, resulting in dementia (1). Characteristic changes in the brain of AD include diffuse loss of neurons, intracellular protein deposits termed neurofibrillary tangles (NFT) consisting of hyperphosphorylated tau protein and extracellular protein deposits termed senile plaques (1). Amyloid β-peptide (Aβ), a major component of senile plaques, is a 39 to 43-amino acid peptide derived by the cleavage of the amyloid precursor protein (2). Several lines of evidence indicate that fibrillar Aβ and, especially, soluble Aβ aggregates are important in the pathophysiology of AD (2). Neurotoxicity mediated by Aβ has been well established in vitro as well as in vivo studies using postmortem brain tissues of AD (1, 2). However, molecular determinants of Aβ-induced neuronal death are still poorly identified.
Gangliosides, sialic acid-containing glycosphingolipids, constitute essential components of the cell membrane and are involved in the modulation of neuronal cell death as well as proliferation and differentiation (3). The disialoganglioside GD3 is highly expressed in embryonic nervous system, declining dramatically with embryonic development and remaining low in the normal adult brain (4). GD3 has attracted attention due to its emerging role as an effector of apoptosis (5). Intracellular GD3 has been reported to increase in response to ceramide, Fas or TNF-α in lymphoid cells and to mediate the early stages of apoptotic process (6-8). It has been reported that Aβ-induced synthesis of GD3 also contributes the development of apoptosis in cortical neurons (9). However, the role of GD3 action in Aβ-induced apoptosis is still unclear. Therefore, this study was undertaken to confirm the changes of GD3 metabolism and to characterize the distribution and trafficking of GD3 during Aβ-induced apoptosis in nerve cells.
Ganglioside mixture and GD3 were purchased from Alexis Co (San Diego, CA, USA) and anti-GD3 monoclonal antibody was from Seikagaku Co (Tokyo, Japan). Synthetic amyloid β-protein fragment 25-35 and anti-mouse µ-chain specific IgM-peroxidase were purchased from Sigma-Aldrich (St. Louis, MO, USA). 5,5',6,6'-Tetrachloro-1,1',3,3'-tetraethylbenzimidazol carbocyanine iodide (JC-1) was obtained from Molecular Probes (Eugene, OR, USA). Annexin V-Fluorescein isothiocynate (FITC) was obtained from BD Biosciences Pharmingen (San Jose, CA, USA). All the other drugs except where indicated were purchased from Sigma (St. Louis, MO, USA).
Human brain-derived TE671 cells were obtained from American Type Culture Collection (ATCC), and maintained with Dulbecco's modified Eagle's medium (DMEM) supplemented with L-glutamine (2 mM), antibiotics (100 U/mL penicillin, 100 mg/mL streptomycin sulfate) and 10% fetal calf serum at 37℃ with 5% CO2 in air atmosphere. At 90% confluence, cells were serum-deprived overnight for the experiments.
Apoptosis was induced by incubating cells with culture medium containing synthetic Aβ fragment (40 µM), GD3 (100 µM) and H2O2 (0.5 mg/mL) for the indicated times. The quantification of apoptotic cells was screened by flow cytometry technique using propidium iodide (PI). Cells were fixed 70% ethanol containing 0.2% Tween 20 and stained with PBS containing PI and RNase. Fluorescence was measured using a FACScan cytometry system (Beckman Coulter Epics XL, Palo Alto, CA, USA). The apoptosis was also quantified by dual-color flow cytometry technique using the annexin V conjugated with Alexa Fluor 488 and PI. Cells were prepared as described above except resuspending in annexin binding reagents for 15 min in the dark without fixation step.
To determine the changes in MMP after treatment with various apoptotic stressors, cells were incubated with culture medium containing JC-1 (1 µM) for 30 min at 37℃, and JC-1 fluorescence was measured by flow cytometry. Mitochondrial integrity was assessed by fluorescence of Mito Tracker Red, and cleavage of caspase-9 and poly (ADP-ribose) polymerase (PARP) were analyzed by western blotting.
Level of cell surface GD3 was measured by flow cytometry. Cells grown on the 6 well plates were washed with ice-cold phosphate buffered saline (PBS), and incubated with PBS containing mouse anti-GD3 antibody (1:100) at 4℃. After washing with PBS, cells were incubated with FITC-labeled goat anti-mouse IgM (1:200). Labeled cells were analyzed using flow cytometry.
To measure the intracellular GD3 levels, cells grown on 10 cm culture dishes were stimulated with various apoptotic stressors and then harvested. Cell pellets were centrifuged and dehydarated by freeze dryer (Eyela FD-1000, Tokyo Rikakikai Co, Japan). To remove neutral lipids, dried cell powder was resuspended in chloroform/methanol (8:2) and sonicated for 30 min at 20℃. Crude gangliosides were extracted from pellet by resuspension with chloroform/methanol/water (2:8:0.5) and sonication. Ganglioside samples were loaded with glass capillary (5 µL) on a silica gel TLC plate (Merck, Darmstadt, Germany) pre-run in chloroform/methanol/0.2% CaCl2 (55:45:10). Authentic GD3 was used as standard. After chromatographed in the same solution, plates were treated with 0.4% poly-iso-butyl-metacrylate in hexane, washed with PBS containing 0.1% Tween 20 and blocked for an hour in PBS with 1% bovine serum albumin. To carry out immunostaining for GD3, the plate was incubated overnight at 4℃ with anti-GD3 monoclonal antibody (1:2,000), followed by incubation for an hour at room temperature with a horseradish peroxidase-conjugated anti-IgM secondary antibody (1:1,000). Specifically stained bands were detected by advanced enhanced chemiluminescence (Amersham Biosciences, Buckinghamshire, UK) with LAS-3000 (Fujifilm, Tokyo, Japan).
Total RNA was extracted using a TRI reagent (Molecular Research Center, Cincinnati, OH, USA). The cDNA was synthesized using 2 µg of RNA and 10 ng/µL of oligo (dT) 15 primer, 1 mM of dNTP, 200 U of M-MLV Reverse Transcriptase (RT) and 1X M-MLV RT buffer (Promega Corporation, Madison, WI, USA). The ABI PRISM® 7000 Sequence Detection System (Applied Biosystems, Foster City, CA,USA) was used for real-time RT-PCR amplification and detection. Real-time RT-PCR was prepared of 25 µL reaction mixture in MicroAmp optical 96-well reaction plates and sealed with optical adhesive covers (Applied Biosystems). Each reaction well contains 1 µL of template DNA, 12.5 µL of SYBR® Green PCR Master Mix (Applied Biosystems, Warrington, UK), and 3 pM of forward and reverse primers. Amplification of GD3 synthase cDNA was performed using the following primers: forward (5'-CCCGAGGAATTTCTCCAACTC-3') and reverse (5'-GCATTCTCAGTGCACCGATTT-3'). Human glyceraldehyde-3-phosphate dehydrogenase (GAPDH) was used as an internal control. Reaction conditions included an initial denaturation step (94℃ for 10 min) followed by 40 cycles of 95℃ for 15 sec, 58℃ for 1 min, and 72℃ for 30 sec. A final extension step (72℃ for 10 min) concluded the reaction.
Cells grown on Lab-Tek 8 well chamber slides were treated with Aβ alone or with methyl-β-cyclodextrin (MβCD) for the indicated time periods. After incubated with Mito Tracker Red for 30 min at 37℃, cells were fixed in 4% paraformaldehyde for 15 min at room temperature, permeabilized with 0.2% Triton X-100 for 15 min and labeled with mouse anti-GD3 antibody alone or in combination with rabbit anti-caveolin-1 antibody 1 hr at room temperature. Cells were washed three times with PBS, and then FITC-conjugated anti-mouse IgM alone or in combination with Texas Red-conjugated anti-rabbit IgG was applied for 45 min at room temperature. After washing three times with PBS, the slides were mounted and examined under the confocal laser scanning microscope (LSM 510, Carl Zeiss, Germany).
Four independent assays were performed. Statistical results were expressed as mean±standard deviation of the means obtained from each independent experiment. The results of the experimental and control groups were tested for statistical significance by a one-tailed Student's t test.
To investigate the capacity of extracellular soluble Aβ to induce apoptosis, human medulloblastoma TE671 cells, which are known to have neuron-like features (10), were treated with 40 µM of Aβ for 24 hr and analyzed by flow cytometry using PI alone or with annexin V-FITC. As shown in Fig. 1, 24 hr treatment of Aβ induced 37% apoptosis. TE671 cells were more susceptible to an apoptosis induced by an oxidative stressor, H2O2 than Aβ. However, extracellular GD3 failed to induce a significant apoptosis in nerve cells after 24 hr treatment at the concentrations up to 100 µM.
To examine whether Aβ-induced apoptosis in TE671 cells is linked to mitochondrial functional damage, mitochondrial changes after Aβ treatment were assessed. Cells were stained with JC-1, a dye sensitive to the changes in mitochondrial membrane potential. As shown in Fig. 2A, control cells showed the typical orange-red fluorescence of J-aggregates at polarized mitochondrial membrane potential. Aβ stimulation for 8 hr to the cells induced increase in green fluorescence of JC-1 monomeric form (0.08% in control; 6.38% in Aβ-treated cells), indicating the collapse of mitochondrial membrane potential. H2O2 treatment caused 19.78% of the cells to lose mitochondrial membrane potential. Aβ-induced mitochondrial damage was further assessed with Mito Tracker Red, a fluorescent mitochondria-specific marker (Fig. 2B). In untreated cells, Mito Tracker was sequestrated in the intact mitochondria appearing as discrete puncta around nucleus. Treatment of Aβ for 24 hr induced Mito Tracker to distribute uniformly in the cytoplasm, indicative of disruption of the mitochondrial integrity. Caspase-9, an important downstream target of cytochrome c release in apoptosome pathway, and poly (ADP-ribose) polymerase (PARP), were activated in cells exposed to Aβ treatment (Fig. 2C). These results show that Aβ-induced apoptosis in nerve cells is linked to mitochondrial damage.
To investigate whether extracellular Aβ induces any change in GD3 metabolism, we analyzed the intracellular level of GD3 in TE671 cells. TLC analysis combined with immunodetection showed a prominent increase of intracellular GD3 content 16 hrs after addition of extracellular Aβ (Fig. 3A). However, no significant increase in intracellular GD3 levels was detected in cells exposed to H2O2.
Because GD3 synthase is responsible for GD3 synthesis from its precursor ganglioside GM3, we examined whether Aβ-induced increase in intracellular GD3 level is related with expression of GD3 synthase. Quantitative real-time RT-PCR revealed that the expression of GD3 synthase mRNA was 14 fold increased at 4 hr of Aβ addition to TE671 cells (Fig. 3B). However, H2O2 treatment did not affect significantly the expression of GD3 synthase mRNA.
Having shown that neosynthesized GD3 is redistributed to nucleus or mitochondria during CD95/Fas- or TNF α-mediated apoptosis (9, 14), we investigated the subcellular trafficking of GD3 during Aβ-induced apoptosis using confocal immunofluorescent study. In untreated basal condition, GD3 staining was negligibly low as a whole except some spots surrounding nucleus and cell periphery (Fig. 3C, D). Interestingly, exposure to extracellular Aβ induced accumulation of GD3 in particular regions of cell surface within an hour (Fig. 3C). Colocalization study showed that GD3 signal in the cell surface was partially overlapped with that of caveolin-1, a membrane caveolae marker. Aβ-induced GD3 accumulation in plasma membrane was peaked at 4 hr, and then dispersed to re-accumulate as clusters of puncta around nucleus after 8 hr of Aβ treatment (Fig. 3D). Punctate pattern of GD3 surrounding nucleus was coincided with that of Mito Tracker. However, trafficking of GD3 to nucleus after Aβ stimulation was not observed in TE671 cells. These findings suggest that neosynthesized GD3 in response to extracellular Aβ accumulates in the plasma membrane before targeting to its final destination, mitochondria.
Accumulation of GD3 in cell surface after Aβ treatment was also confirmed by flow cytometry analysis. Fig. 3E shows that the cell surface GD3 level increased at 4 hr after Aβ addition, whereas H2O2 treatment did not induce any significant change.
Finally, we investigated whether perturbation of GD3 accumulation in cell surface and its targeting to mitochondria could prevent Aβ-induced apoptosis. Experiments were carried out by preincubating cells with MβCD to deplete membrane lipid microdomains of either plasma membrane or mitochondrial membrane. As shown in Fig. 4A, MβCD treatment resulted in round cell morphology with loss of caveolin-1-postive puncta along the cell periphery. Both immunofluorescent microscopy and flow cytometry analysis revealed that MβCD blocked Aβ-induced GD3 accumulation in plasma membrane (Fig. 4A, B). The apoptosis rates of TE671 cells after Aβ treatment were approximately 22% at 4 hr (data not shown) and 37% at 24 hr (Fig. 4C), respectively. Interestingly, treatment of MβCD resulted in 41% reduction of Aβ-induced apoptosis after 24 hr. On the contrary, H2O2-induced apoptosis was rather increased with MβCD treatment. These findings suggest that GD3 accumulation, which is mainly determined by both its delivery from Golgi complex, the site of synthesis, to plasma membrane and the exit from plasma membrane to mitochondria via lipid microdomains, plays a key role in Aβ-induced apoptosis.
We showed here that extracellular Aβ induces GD3 synthase expression to increase intracellular GD3 level and that neosynthesized GD3 accumulates in plasma membrane microdomains before it is translocated to mitochondria to induce mitochondrial damage in TE671 cells. We also demonstrated that blocking GD3 accumulation and trafficking in cell surface by MβCD significantly prevents Aβ-induced apoptosis, suggesting that cell surface GD3 acts as an important step in Aβ-induced apoptosis.
GD3 has attracted considerable attention due to its role as a cell death effector (6, 11). Increased intracellular GD3 are known to be required in CD95- and ceramide-induced apoptosis in hematopoietic cells (6) as well as TNF-α-induced apoptosis in hepatocytes (12) and colon cells (11). GD3 activation has also been reported to involve in Aβ-induced apoptosis in cortical neurons (9).
There are accumulating evidences that GD3 acts as a core intracellular mediator inducing dissipation of the inner mitochondrial transmembrane potential through opening the permeability transition pore complex (PTPC) and the release of mitochondrial proapoptogenic factors such as cytochrome c, ATP, apoptosis inducing factor and mitochondrial caspases (13-15). GD3 also elicits a burst of reactive oxygen species production from mitochondrial respiratory chain, contributing the apoptosome activation (16). However, the exact target of GD3 in mitochondria still remains to be elucidated. Interestingly, apoptotic potential of GD3 is known to be modulated by acetylation and oxidation. Acetylated GD3, especially 9-O-acetyl GD3, is unable to elicit, rather suppresses proapoptotic activity of GD3 (17), whereas the main product of GD3 oxidation, GD3-7-aldehyde, is more efficient to induce PTPC-dependent apoptosis than GD3 in HL-60 cells (18).
Gangliosides are distributed predominantly in the plasma membrane and the early Golgi compartment. GM1 and GM3 are known to be the abundant gangliosides in membrane lipid microdomains whereas GD3 is a minor ganglioside in most normal tissues (19). Gangliosides are synthesized through several steps occurring in different intracellular compartments (15). The carbon backbone of gangliosides, ceramide, is either de novo synthesized in the endoplasmic reticulum or arises from hydrolysis of sphingomyelin-engaging sphingomyelinases (SMases). Stepwise glucosylation and galactosylation of ceramide occurs in the Golgi, producing lacosylceramide. Sequential addition of sialic acids to lacosylceramide results in the formation of gangliosides. The α 2,8-sialyl-transferase, called GD3 synthase, is known to reside in early Golgi and catalyzes the addition of a second sialic acid to GM3 to generate GD3, one of b-series of gangliosides (5). In our study, extracellular Aβ induced marked expression of GD3 synthase mRNA (Fig. 3). Although it remains to be determined whether extracellular Aβ induces GD3 synthase through death receptors in cell membrane, GD3 synthase expression is responsible to the increased intracellular GD3 after Aβ treatment. As ceramide synthesized by acidic SMase contributes GD3 generation in TNF-α-mediated apoptosis (11), it is worthwhile to evaluate if extracellular Aβ induces acidic SMase as well. Sialidases (Neu), key enzymes in the degradation of gangliosides, also affect the GD3 concentration in membranes (20). It has been demonstrated that the expression level of Neu4 sialidase dramatically decreases with GD3 accumulation in mitochondria prior to neuronal apoptosis induced by catechol metabolites (21).
Aβ binding and aggregation occurs in the presence of plasma membrane lipids, especially cholesterol and gangliosides (22). Lipid rafts are specialized membrane domains where numerous receptors with their downstream signaling molecules are concentrated (23). This microdomain has higher concentrations of cholesterol and gangliosides than any other membrane substructures (24). Several studies have demonstrated that soluble extracellular Aβ interacts with gangliosides in the outer leaflet of cholesterol-rich lipid rafts to form toxic Aβ aggregation (25). In fact, GM1-bound Aβ has been found in brains exhibiting early pathologic changes of Alzheimer's disease (26). We demonstrated here that GD3, which was not detected in basal condition, increased with exogenous Aβ and distributed unevenly along the cell margin as puncta of different sizes with partial colocalization with caveolin-1 (Fig. 3). This finding suggests that neosynthesized GD3 localizes in lipid raft microdomains including caveolae. Several studies have also shown that GD3 localizes constitutively in plasma membrane lipid rafts in rat cerebellar granular neurons and hepatocytes (12, 27). However, in our study, the GD3 level in lipid rafts is not noticeable at basal condition, rather induced with extracellular Aβ. Whether GD3 in lipid rafts is derived from hydrolysis of sphingomyelin in situ or de novo synthesis in the Golgi during Aβ-induced apoptosis needs to be determined.
Increased GD3 in plasma membrane lipid microdomains might facilitate the conformation changes of soluble extracellular Aβ into toxic Aβ aggregation, which triggers neuronal apoptosis. Recently, it has been reported that primary neurons and astrocytes isolated from GD3 synthase knockout mice exhibited resistance to Aβ-induced cell death and Aβ aggregation (28), showing the requirement of GD3 and its synthase in Aβ aggregation and Aβ-induced apoptosis. We also demonstrated here that cholesterol depletion in lipid rafts by MβCD prevented not only GD3 increase in cell surface but also Aβ-induced apoptosis (Fig. 4). These findings, in agreement with other reports, underline the importance of GD3 in lipid microdomains of cell membranes in Aβ-triggered apoptosis.
Copani et al. (9) first reported that GD3 activation involved in Aβ-induced apoptosis by reactivating unscheduled cell cycle to enter into S-phase in cortical neurons. They showed that neosynthesized GD3 accumulated inside the nuclear region, speculating that GD3 provides nuclear signal for aberrant DNA replication. Several other studies have also reported that GD3 is translocated to nucleus and acts as a repressor of the TNF-inducible NF-κB-dependent induction of survival genes in hepatocytes (29) and induce post-translational modification of histone H1 during anti-CD95 antibody-triggered apoptosis in HUT-78 cells (30). However, in our experiments using brain-derived TE671 cells, nuclear accumulation of GD3 was not observed during Aβ-induced apoptosis. Rather, GD3 prominently accumulated in plasma membrane before mitochondrial targeting.
In summary, our data suggest that rapidly accumulated GD3 in plasma membrane lipid microdomains, prior to mitochondrial translocation in response to extracellular Aβ, might be one of the key events in Aβ-induced apoptosis. Inhibition of Aβ-induced apoptosis through modulating GD3 accumulation in lipid rafts and its trafficking to mitochondria might be a novel therapeutic strategy in neurodegenerative diseases related with Aβ pathology.
Figures and Tables
Fig. 1
Amyloid β-induced apoptosis. TE671 cells were treated with GD3 (100 µM), Aβ (40 µM) or H2O2 (0.5 mg/mL) for 24 hr. Apoptotic cell death was quantified by flow cytometry analysis using PI alone (A) or with annexin V-FITC (B). Results are given as the mean±S.D. of four individual experiments (C).
*P<0.01.

Fig. 2
Mitochondrial involvement in amyloid β-induced apoptosis. (A) TE671 cells were exposed to GD3 (100 µM), Aβ (40 µM) or H2O2 (0.5 mg/mL) for 8 hr. Dissipation of mitochondrial membrane potential was assessed by shift of JC-1 aggregates from red to green fluorescence using flow cytometry. Numbers represent percent of cells in the lower right quadrant. (B) Mitochondrial integrity was evaluated using Mito Tracker Red after 24 hr of Aβ treatments. (C) Caspase 9 and PARP activation after Aβ treatment were assessed by western blotting using cells lysates.

Fig. 3
GD3 synthesis and trafficking during amyloid β-induced apoptosis. (A) TE671 cells were treated with either Aβ (40 µM) or H2O2 (0.5 mg/mL) for 16 hr. Intracellular GD3 levels were measured by thin layer chromatography immunostaining using the crude ganglioside cell extracts. (B) Expression of GD3 synthase after 4 hr of Aβ treatment was analyzed by real-time quantitative RT-PCR using GAPDH as an internal control. Results are given as the mean±S.D. of four individual experiments. *P<0.01. (C) Localization of intracellular GD3 after Aβ treatment was evaluated by double immunofluorescence staining technique with FITC-labeled anti-GD3 and Texas Red-labeled anti-caveolin-1 antibodies. (D) Cells were loaded with Mito Tracker Red for 30 min before fixation and then labeled with FITC-conjugated anti-GD3 antibody. (E) Cell surface GD3 levels after 4 hr of Aβ treatment were analyzed by flow cytometry using FITC-labeled anti-GD3 antibody.
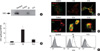
Fig. 4
Blockage of amyloid β-induced apoptosis by preventing cell surface GD3 accumulation and trafficking. (A) TE671 cells were pretreated with methyl-β-cyclodextrin (0.5 mg/mL) for 1 hr and then exposed to Aβ (40 µM) for 4 hr. Localization of intracellular GD3 was evaluated by double immunofluorescence staining technique with FITC-labeled anti-GD3 and Texas Red-labeled anti-caveolin-1 antibodies. (B) Cell surface GD3 levels after 4 hr of Aβ treatment were analyzed by flow cytometry using FITC-labeled anti-GD3 antibody. (C) Apoptotic cell death was quantified after 24 hr of Aβ treatment by flow cytometry analysis using PI. Results are given as the mean±S.D. of four individual experiments. *P<0.05.

References
2. Haass C, Selkoe DJ. Soluble protein oligomers in neurodegeneration: lessons from the Alzheimer's amyloid β-peptide. Nat Rev Mol Cell Biol. 2007. 8:101–112.


3. Hakomori S. Glycosylation defining cancer malignancy: New wine in an old bottle. Proc Natl Acad Sci USA. 2002. 99:10231–10233.


4. Lee MC, Lee WS, Park CS, Juhng SW. The biologic role of ganglioside in neuronal differentiation: effects of GM1 ganglioside on human neuroblastoma SH-SY5Y cells. J Korean Med Sci. 1994. 9:179–187.


6. De Maria R, Lenti L, Malisan F, d'Agostino F, Tomassini B, Zeuner A, Rippo MR, Testi R. Requirement for GD3 ganglioside in CD95- and ceramide-induced apoptosis. Science. 1997. 277:1652–1655.


8. Simon BM, Malisan F, Testi R, Nicotera P, Leist M. Disialoganglioside GD3 is released by microglia and induces oligodendrocyte apoptosis. Cell Death Differ. 2002. 9:758–767.


9. Copani A, Melchiorri D, Caricasole A, Martini F, Sale P, Carnevale R, Gradini R, Sortino MA, Lenti L, De Maria R, Nicoletti F. β-Amyloid-induced synthesis of the ganglioside GD3 is a requisite for cell cycle reactivation and apoptosis in neurons. J Neurosci. 2002. 22:3963–3968.


10. Synapin PJ, Salvaterra PM, Engelhardt JK. Neuron-like features of TE671 cells: presence of a functional nicotinic cholinergic receptor. Brain Res. 1982. 231:365–377.
11. Colell A, Morales A, Fernandez-Checa JC, Garcia-Ruiz C. Ceramide generated by acidic sphingomyelinase contributes to tumor necrosis factor-alpha-mediated apoptosis in human colon HT-29 cells through glycosphingolipids formation. Possible role of ganglioside GD3. FEBS Lett. 2002. 526:135–141.
12. Garcia-Ruiz C, Colell A, Morales A, Calvo M, Enrich C, Fernández-Checa JC. Trafficking of ganglioside GD3 to mitochondria by tumor necrosis factor-alpha. J Biol Chem. 2002. 277:36443–36448.
13. Kristal BS, Brown AM. Apoptogenic ganglioside GD3 directly induces the mitochondrial permeability transition. J Biol Chem. 1999. 274:23169–23175.


15. Morales A, Colell A, Mari M, Garcia-Ruiz C, Fernandez-Checa JC. Glycosphingolipids and mitochondria: role in apoptosis and disease. Glycoconj J. 2004. 20:579–588.


16. Garcia-Ruiz C, Colell A, Paris R, Fernandez-Checa JC. Direct interaction of GD3 ganglioside with mitochondria generates reactive oxygen species followed by mitochondrial permeability transition, cytochrome c release, and caspase activation. FASEB J. 2000. 14:847–858.
17. Malisan F, Franchi L, Tomassini B, Ventura N, Condo I, Rippo MR, Rufini A, Liberati L, Nachtigall C, Kniep B, Testi R. Acetylation suppresses the proapoptotic activity of GD3 ganglioside. J Exp Med. 2002. 196:1535–1541.


18. Brenner C, Kniep B, Maillier E, Martel C, Franke C, Röber N, Bachmann M, Rieber EP, Sandhoff R. GD3-7-aldehyde is an apoptosis inducer and interacts with adenine nucleotide translocase. Biochem Biophys Res Commun. 2010. 391:248–253.


19. Ariga T, McDonald MP, Yu RK. Role of ganglioside metabolism in the pathogenesis of Alzheimer's disease - a review. J Lipid Res. 2008. 49:1157–1175.
20. Miyagi T, Wada T, Yamaguchi K, Hata K, Shiozaki K. Plasma membrane-associated sialidase as a crucial regulator of transmembrane signaling. J Biochem. 2008. 144:279–285.
21. Hasegawa T, Sugeno N, Takeda A, Matsuzaki-Kobayashi M, Kikuchi A, Furukawa K, Miyagi T, Itoyama Y. Role of Neu4L sialidase and its substrate ganglioside GD3 in neuronal apoptosis induced by catechol metabolites. FEBS Lett. 2007. 581:406–412.


22. Choo-Smith LP, Garzon-Rodriguez W, Glabe CG, Surewicz WK. Acceleration of amyloid fibril formation by specific binding of Abeta-(1-40) peptide to ganglioside-containing membrane vesicles. J Biol Chem. 1997. 272:22987–22990.
24. Patron RG. Ultrastructural localization of gangliosides; GM1 is concentrated in caveolae. J Histochem Cytochem. 1994. 42:155–166.
25. Kakio A, Nishimoto S, Kozutsumi Y, Matsuzaki K. Formation of a membrane-active form of amyloid β-protein in raft-like model membranes. Biochem Biophys Res Commun. 2003. 303:514–518.


26. Hayashi H, Kimura N, Yamaguchi H, Hasegawa K, Yokoseki T, Shibata M, Yamamoto N, Michikawa M, Yoshikawa Y, Terao K, Matsuzaki K, Lemere CA, Selkoe DJ, Naiki H, Yanagisawa K. A seed for Alzheimer amyloid in the brain. J Neurosci. 2004. 24:4894–4902.


27. Vyas KA, Patel HV, Vyas AA, Schnaar RL. Segregation of gangliosides GM1 and GD3 on cell membranes, isolated membrane rafts, and defined supported lipid monolayers. Biol Chem. 2001. 382:241–250.


28. Bernardo A, Harrison FE, McCord M, Zhao J, Bruchey A, Davies SS, Jackson Roberts L 2nd, Mathews PM, Matsuoka Y, Ariga T, Yu RK, Thompson R, McDonald MP. Elimination of GD3 synthase improves memory and reduces amyloid-beta plaque load in transgenic mice. Neurobiol Aging. 2009. 30:1777–1791.
29. Colell A, Garcia-Ruiz C, Roman J, Ballesta A, Fernandez-Checa JC. Ganglioside GD3 enhances apoptosis by suppressing the nuclear factor-kappa B-dependent survival pathway. FASEB J. 2001. 15:1068–1070.