Abstract
Connective tissue growth factor (CTGF) is known to be a profibrotic growth factor, which mediate the fibrotic effect of transforming growth factor-β (TGF-β) and to stimulate cell proliferation and matrix production. CTGF has been shown to be hypoxia-inducible in several cell types. Here we investigated the effect of hypoxia on CTGF gene expression in cultured mouse renal tubular cells (MTC). Quiescent cultures of MTC were exposed to hypoxia (1% O2) or normoxia in serum-free medium. The effects on hypoxia-induced CTGF expression were evaluated by Northern blot and real-time PCR. The roles of mitogen-activated protein kinase (MAPK) and TGF-β were also determined using specific biochemical inhibitors. Exposure of quiescent tubular cells to hypoxia for 24 hr in a conditioned medium resulted in a significant increase TGF-β. Hypoxia caused a significant increase in CTGF mRNA expression in MTC. Either JNK or ERK inhibitor did not block the hypoxia-induced stimulation of CTGF, whereas an inhibitor of p38 MAPK reduced the hypoxia-induced changes of CTGF. Although hypoxia stimulated TGF-β production, neutralizing anti-TGF-β1 antibody did not abolish the hypoxia-induced CTGF mRNA expression. The data suggest that hypoxia up-regulates CTGF gene expression, and that p38 MAPK plays a role in hypoxic-stimulation of CTGF. We also demonstrated that hypoxia induces CTGF mRNA expression via a TGF-β1-independent mechanism.
Recent studies suggest the role of hypoxia in the tubulointerstitium as a common final pathway to end-stage renal disease (1-3). Hypoxia has been shown to induce cellular proliferation and extracellular matrix (ECM) synthesis by cultured mesangial cells (4, 5) and fibroblasts (6). Hypoxia has also been shown to increase ECM synthesis in renal tubular epithelial cells as well as inducing epithelial-to-mesenchymal transdifferentiation (EMT) and apoptosis (1, 7). Chronic renal hypoxia may occur by changes of oxygen supply (hypoxic injury) or impairment of blood flow to tubulointerstitium. Histologic studies of animal model and human kidney suggest that insufficient oxygenation resulting from peritubular capillary loss has a pivotal role in the pathogenesis of renal disease (8, 9). But, the underlying signaling mechanisms whereby hypoxia alters cellular behaviors remain poorly defined. Hypoxia can regulate expressions of a variety of growth factors and cytokines in a cell and tissue-specific manner. These include transforming growth factor-β (TGF-β), vascular endothelial growth factor (VEGF), and endothelin-1 (ET-1) (10).
Connective tissue growth factor (CTGF) is a 38 kD cysteine-rich heparin-binding protein belonging to the CCN family and involves in stimulation of proliferation, angiogenesis, migration, ECM production, cell attachment, cell survival and apoptosis (11). TGF-β enhances CTGF mRNA and protein expression in mesangial cells (12). CTGF has been proposed to play an important role in tubulointerstitial fibrosis as one of the major mediators of TGF-β. It has been shown to be hypoxia-inducible in human breast cancer cells (13). Higgins et al. were the first to show hypoxic induction of CTGF in renal tubular epithelial cell cultures (14). But, the precise signaling mechanisms of the hypoxia-induced expression of CTGF remain unclear.
In the present study, we investigated: 1) the effect of hypoxia on TGF-β concentration in conditioned medium and CTGF gene expression in cultured renal tubular cells; 2) what kind of mitogen-activated protein (MAP) kinase is involved in hypoxia-stimulated CTGF mRNA expression; and 3) whether hypoxia-induced CTGF mRNA expression is mediated by the release of TGF-β1.
Mouse tubular cells (MTC) are a proximal epithelial cell line isolated from 8-10-week-old naïve SJL/J (H-2s) mice as previously described (15) and cultured in renal epithelial cell basal medium (REBM) (Clonetics, San Diego, CA, U.S.A.) containing 5% fetal bovine serum (FBS), 5,000 U/mL penicillin, 5,000 µg/mL streptomycin, and L-glutamine. Cultures were maintained in 75 cm3 flasks at 37℃ under a humidified atmosphere of 5% CO2/95% air. Cells were passed by tripsinization after they reached 80% confluency and utilized between passages three and seven for all of the studies. Confluent cells were made quiescent by 24 hr incubation in serum-free medium.
Fresh quiescence medium was added and cells were exposed to 1% O2, 4% CO2, 95% N2 in an Anaerobic system 1,029 (Forma Scientific, Marietta, OH, U.S.A.) in open dishes. Cells were overlaid with medium to a depth of 3 mm sufficient to prevent dehydration. Control dishes were incubated for equivalent periods under normoxic conditions (21% O2, 5% CO2, 37℃). The tetrazolium dye-reduction assay (MTT; 3-[4,5-dimethylthiazol-2-yl]-2,5-diphenyl tetrazolium bromide; Sigma) was used to test cell viability before and at end of treatment and did not reveal any signs of increased cell death in hypoxia (data not shown).
To determine the effect of hypoxia on CTGF gene expression, MTC were grown in hypoxic condition for up to 10 hr. At the end of each incubation time, cultures were harvested and total cellular RNA was extracted using TRIZOLR Reagent (Gibco-BRL, MD, U.S.A.) according to the manufacturer's instructions. Thirty micrograms of total RNA were electrophoresed on a 1.2% formaldehyde-agarose gel and ethidium-bromide-stained gels were photographed under UV illumination. RNA was transferred to nitrocellulose membranes for 3 hr. Membranes were prehybridized for 1 hr at 68℃ in Quick Hyb Buffer (Stratagene, La Jolla, CA, U.S.A.) and hybridized for 3 hr in the same solution containing the 32P-labeled cDNA probes for human CTGF at 68℃. The blots were then washed two times, 30 min each in 4×SSC, 0.1% SDS at 68℃ followed by three 1 hr washes with 0.2×SSC, 0.1% SDS at 55℃. The blots were exposed to Kodak XAR-5 film (Eastman Kodak, Rochester, NY, U.S.A.). Photographs of ethidium bromide-stained gels and autoradiograms were scanned and quantified by densitometry. To control for relative equivalence of RNA loading, the blots were hybridized with β-actin cDNA (Clothech) probe or an oligonucleotide probe corresponding to the 18S rRNA. Data are expressed as relative mRNA levels calculated as a relative ratio of control value from arbitrary densitometry units.
CTGF mRNA transcripts were detected by a real-time quantitative real-time PCR procedure using ABI Prism 7000 Sequence Detection System (PE Applied Biosystems, Foster City, CA, U.S.A.). This system is based on the ability of the 5' nuclease activity of Taq polymerase to cleave a dual-labeled fluorogenic hybridization probe during DNA chain extension. The probe is labeled with a reporter fluorescent dye FAM at the 5' end and a quencher fluorescent dye TAMRA (6-carboxy-tetramethyl-rhodamine) at the 3' end. During the extension phase of PCR, the nucleolytic activity of the DNA polymerase cleaves the hybridization probe and releases the reporter dye from the probe with concomitant increase in reporter fluorescence. The following sequence-specific primers and probes were designed using Primer Express software (PE Applied Biosystems, Foster City, CA, U.S.A.): Rat CTGF forward primer: 5'-CAA GCT GCC CGG GAA AT-3', reverse primer: 5'-CGG TCC TTG GGC TCA TCA-3', and probe: 5'FAM-CTG TGA GGA GTG GGT-MGBNFQ-3'; 18S rRNA forward primer 5'-CGG CTA CCA CAT CCA AGG AA-3', reverse primer: 5'-GCT GGA ATT ACC GCG GCT-3': probe 5'VIC-TGC TGG CAC CAG ACT TGC CCT C-TAMRA 3'. Primers were used at a concentration of 900 nM and probes at 100 nM at each reaction. Reactions were assembled in a 96-well optical reaction plate. Each reaction contained 1×master mix from the kit (TaqMan One-Step RT-PCR Master Mix; Applied Biosystems, Inc.), forward primer, reverse primer, fluorescent probe, and RNA sample to a final volume of 25 µL per reaction. The plate was analyzed on a sequence-detection system, which simultaneously performs RT-PCR and detects fluorescence signal.
The cell supernatant was collected at each time point, clarified by centrifugation at 1,500×g for 5 min at 4℃ and stored at -80℃. Release of TGF-β1 into culture supernatant of cells was measured using corresponding ELISA kits according to the manufacturer's instructions (R&D Systems, Minneapolis, MN, U.S.A.). As the ELISA only measured levels of the active protein, activation of the supernatant was performed to measure the total levels of both proteins. Briefly, cell supernatants were activated with 1.0 N HCl and subsequently neutralized with 1.2 N NaOH/0.5 M HEPES. Acid-activated samples were added to the precoated microplates and incubated at room temperature for 2 hr. A second antibody, anti-TGF-β1 polyclonal antibody, was then added followed by TGF-β1 horseradish peroxidase conjugate. TGF-β1 was detected by adding the chromogenic substrate and this was followed by stop solution. Absorbance was determined at optical density 450 nm.
We also examined the role of MAP kinase in stimulation of CTGF mRNA induced by hypoxia. Cells were pretreated with MAP kinase inhibitors for 30 min, and incubated under hypoxic or normoxic conditions for 4 hr. Inhibitors were: PD-098059, SB-203580 and JNK inhibitor (all from Calbiochem, La Jolla, CA, U.S.A.). At the end of incubation, the hypoxia-induced CTGF expression was evaluated by Northern blot and RT-PCR.
To determine whether the effects of hypoxia on CTGF gene expression are mediated by TGF-β1, anti-TGF-β1 antibody (2 µg/mL; R&D Systems, Minneapolis, MN, U.S.A.) was added to cells for 30 min immediately before hypoxia. Parallel experiments were undertaken without anti-TGF-β1 antibody. Levels of CTGF mRNA expression were measured by RT-PCR as described above.
We then examined the effect of hypoxia on CTGF gene expression in MTC. Quiescent cultures were exposed in serum-free medium to hypoxia for 2, 3, 4, 6, 8, or 10 hr. At the end of each incubation time, CTGF gene expression was assessed by Northern blot analysis. As shown in Fig. 1, hypoxia caused a significant increase in CTGF mRNA expression in MTC. The steady-state level of CTGF mRNA was maximally up regulated by 3-fold within 4 hr as compared with the cells cultured under the normoxic condition. Subsequently, there was a decline, but a 2-fold elevated level was maintained for 10 hr.
Since TGF-β has been reported to activate the MAP kinase pathway (16-18), we tested the possible participation of MAP kinases in hypoxia-induced CTGF expression. We examined the activation of MAP kinase in response to hypoxia over 4-hr period. To inhibit MAP kinase, ERK inhibitors (PD-098059, 50 µM/L), p38 inhibitor (SB-203580, 20 µM/L) or JNK inhibitor (20 µM/L) was incubated 30 min before hypoxic stimulation. The effects of MAP kinase on hypoxia-induced CTGF gene expression were studied by RT-PCR (Fig. 2). Interestingly, ERK, p38 and JNK showed a different response to hypoxia on CTGF mRNA expression. Either JNK or ERK inhibitor did not block the up-regulation by hypoxia of CTGF expression, whereas an inhibitor of p38 MAP kinase reduced the hypoxia-induced stimulation of CTGF.
TGF-β has been shown to increase the expression of CTGF by a variety of cells (12, 19, 20). As a foundation for defining the TGF-β1 regulating CTGF expression in our system, we first determined the response of cultured renal tubular cells to TGF-β1. Quiescent cultures of MTC were exposed to normoxic condition in serum-free medium, and then treated with 10 ng/mL TGF-β1 for 8 hr. A time-course study of the response demonstrated that there was a little delay before the level of CTGF mRNA began to increase after the addition of TGF-β1 (Fig. 3). TGF-β1 induced the expression of CTGF mRNA that began within 30 min, peaked at 2 hr and then declined by 8 hr.
TGF-β1 is the major fibrogenic cytokine within the kidney and induced by hypoxia in a variety of cell types. Concentrations of TGF-β1 by MTC was examined using ELISA to measure TGF-β1 in the cell supernatant (Fig. 4). Hypoxia induced a 91% increase in the amount of TGF-β1 after 4 hr of hypoxia: 30.14±13.30 ng/mL vs. 15.74±2.92 ng/mL in control. Assay of the cell supernatant in the 24-hr hypoxia showed a 161% increase in the amount of TGF-β1 (41.16±6.31 ng/mL).
Since CTGF is an response gene to TGF-β stimulation, we tested whether TGF-β synthesis was required for the enhanced expression of CTGF by hypoxia, MTC were exposed to hypoxia for 4 or 8 hr in the presence of anti-TGF-β1 antibody (2 µg/mL) to block TGF-β produced by the cells after hypoxia. The results showed that hypoxia produced substantial increases in the level of CTGF mRNA in both the absence and the presence of anti-TGF-β1 antibody (Fig. 5). These findings suggested that TGF-β1 synthesis was not required for hypoxia-induced stimulation of CTGF and TGF-β1 is not the primary mediator of the hypoxia-induced CTGF gene expression.
In this study, we found that hypoxia stimulated TGF-β1 and CTGF expression in cultured renal tubular cells. We also demonstrated that either JNK or ERK inhibitor did not block hypoxia-mediated CTGF expression, whereas an inhibitor of p38 MAP kinase reduced the expression of CTGF. Although hypoxia stimulated TGF-β production, neutralizing anti-TGF-β1 antibody did not abolish the hypoxia-induced CTGF mRNA expression. These data suggest that hypoxia regulates CTGF expression through TGF-β1-independent mechanism.
A number of studies have shown that hypoxia leads to profibrotic responses in tubular epithelial cells and renal fibroblasts (2, 5, 6). Since tubular hypoxia is present in the early stage of disease in a progressive glomerulonephritis model (8), hypoxia from peritubular capillary loss may contribute to the process of renal fibrosis. Although numerous signaling pathways have been implicated in hypoxic signal transduction, the specific mechanisms underlying the fibrogenic response to hypoxia remain unknown. These could be mediated by direct effects on gene expression via hypoxia response elements (HRE) present in the gene promoters or secondary factors induced by hypoxia (14, 21). At the center of this cellular response to hypoxia is hypoxia-inducible factor (HIF) (22, 23). HIF is composed of two subunits, an oxygen-sensitive HIF-a subunit and a constitutively expressed HIF-b subunit (1). Hypoxia also modulates the activation of Nuclear factor-kappaB (NF-κB) in cells through decreased oxygen-dependent suppression of the key regulators of this pathway (24). Hypoxia has been reported to stimulate the production of a variety of growth factors including the profibrotic factor, TGF-β, in multiple cell types (10, 25, 26). In our study, hypoxic treatment produced a 2- to 3-fold increase in the level of TGF-β1 in the cell supernatant. The period of hypoxia in this study (24 hr, 1% O2) was based on data showing that in vitro exposure of various cell lines to 1% O2 for a minimum of 16 hr alters gene expression (25). Although previous studies showed that 24 hr of hypoxia induced a increase in the level of TGF-β1 (27), we have shown the shorter period of hypoxia also increased TGF-β1 production.
CTGF has recently received much attention as a key determinant of progressive fibrosis and also wound repair, neoangiogenesis, bone formation and embryonic development (28). Comparison of the mouse and rat CTGF proteins showed a 95% identity and the rat CTGF protein also showed 91% identity to the human CTGF protein (29). TGF-β induces CTGF through different signaling pathways and a specific TGF-β responsive element in the CTGF promotor (12). CTGF is thought to act both as a profibrotic marker and as a downstream mediator of TGF-β. In the present cell culture system, TGF-β1 produced a 15-fold increase in the level of CTGF mRNA. The induction of CTGF mRNA by TGF-β1 was also found in renal fibroblasts (unpublished observations). These results are consistent with observations in other cell systems where TGF-β induces CTGF expression (12, 19, 20). It has previously been found that CTGF was regulated by hypoxia in a human breast cancer cell line, MDA231 (13). Our present study demonstrated that hypoxic treatment lead to significant elevation of CTGF mRNA in renal tubular cells. But, we have not measured CTGF protein levels. The steady-state level of CTGF mRNA in MTC was maximally up-regulated by 3-fold within 4 hr as compared with that from the normoxic condition. Subsequently, there was a decline, but a 2-fold elevated level was maintained for 10 hr. Similarly, hypoxia (0.5% O2) has been shown to stimulate CTGF mRNA by 2-fold in renal tubular epithelial cells (14).
Since transcription is the major level of modulation of CTGF expression by TGF-β1 and since TGF-β has been reported to activate the MAP kinase pathway (16-18), we tested what kind of kinase was involved in hypoxia-induced CTGF expression. We found distinct difference in the role of MAP kinases in mediating CTGF expression in response to hypoxia. An inhibitor of p38 MAP kinase (SB-203580) reduced hypoxia-stimulated CTGF expression, whereas either JNK or ERK inhibitor (PD-098059) did not block the expression of CTGF. The roles of MAP kinases under hypoxia are complicated, which depend on the specific cell types. ERK activation has been shown to be critical in hypoxia-induced VEGF expression in HepG2 cells (30). In contrast, the study using NRK52 cells, ERK activation was not observed in response to hypoxia, whereas activation of p38 could be demonstrated (26). In terms of p38, several studies have reported its critical role under hypoxia (26, 31), which is compatible with our data. To our knowledge, the roles of MAP kinases in CTGF regulation of renal tubular cells in response to hypoxia have not been previously examined. Therefore the one new finding is that hypoxia induces the expression of CTGF in a p38 MAPK-dependent manner. Demonstration of different roles of MAP kinases under hypoxic condition would be important in the investigation of the mechanisms regulating CTGF expression.
The present work has focused on TGF-β as a mediator to increase the expression of CTGF gene by hypoxia in cultured renal tubular cells. Since CTGF acts as a mediator of TGF-β1 signaling, we hypothesized that hypoxia-induced TGF-β contributes to CTGF expression. Although TGF-β1 activity increased in response to hypoxia, the addition of the neutralizing anti-TGF-β1 antibody did not block the hypoxia-induced CTGF expression. These data suggest that TGF-β1 is not the primary mediator of the hypoxia-induced CTGF expression in renal tubular cells. Also, hypoxia-induced CTGF expression seems not to be from the increased bioactivity of TGF-β1. Our data examining signaling pathways involved in CTGF gene expression are in good agreement with some recent studies. Recent study showed that TGF-β1 signaling was not required for hypoxic induction of CTGF promotor activity in renal tubular cells (14). The mechanisms by which hypoxia stimulates CTGF gene expression remain to be worked out but appear to be independent of TGF-β1. Limitations of our study were that the level of CTGF protein and in particular secreted protein were not measured, while mRNA expression was important.
In summary, this study demonstrates that hypoxia up-regulates CTGF gene expression in cultured tubular cells and hypoxia-induced CTGF activation is dependent on p38 MAP kinase. Based on our study on CTGF gene regulation, we have identified that hypoxia regulates CTGF expression through TGF-β1-independent pathway. The data suggest an important role of CTGF in fibrogenic effect by hypoxia. Understanding the molecular mechanisms by which hypoxia induced fibrosis may open new avenues to the treatment of progressive renal diseases.
Figures and Tables
Fig. 1
Time course of CTGF mRNA expression in response to hypoxia. MTC were isolated, cultured until 80% confluent, and exposed to 1% oxygen for 0-10 hr. CTGF transcripts were detected by Northern blot analysis; a representative blot is shown along with ethidium bromide stained 28S and 18S rRNA for loading. Densitometric analysis for CTGF mRNA levels is shown normalized to 18S rRNA level. Data are representative of three separate experiments.
*p<0.05 compared with 0 hr sample.
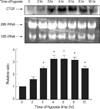
Fig. 2
Effects of MAPK inhibitors on hypoxia-induced CTGF expression. Quiescent cells were pretreated for 30 min either with PD-098059 (PD, 50 µM/L), SB-203580 (SB, 20 µM/L), or JNK inhibitor (20 µM/L) followed by exposure to hypoxia or normoxia for 4 hr. At the end of incubations, total RNA was prepared from the cells and subjected to and RT-PCR. *p<0.05 compared with hypoxia.
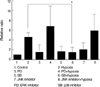
Fig. 3
TGF-β1 stimulation of CTGF in normoxic condition. TGF-β1 induces CTGF mRNA in cultured MTC. Tubular cells were serum starved for 24 hr before being treated with 10 ng/mL TGF-β1. Cells were harvested at various time points and RNA was extracted. Northern hybridization for CTGF mRNA levels were performed and normalized against endogenous 18S ribosomal RNA. *p<0.05 compared with 0 hr sample.
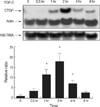
Fig. 4
Effect of hypoxia on levels of TGF-β1 in MTC. MTC were isolated, cultured until 80% confluent, and exposed to 1% oxygen for 0-24 hr. Conditioned medium was collected at the end of hypoxia. TGF-β level in the cell medium was measured by a TGF-β ELISA kit. *p<0.05 compared with 0 hr sample.
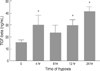
Fig. 5
Effects of anti-TGF-β1 antibody on CTGF activity exposed to hypoxia. MTC were exposed to hypoxia in the presence of 2 µg/mL of anti-TGF-β1 antibody for 30 min, followed by exposure to hypoxia for 0-8 hr. Relative CTGF levels were calculated as a percentage of the relevant control values from arbitrary densitometry units. NS, not significant.
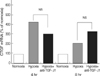
ACKNOWLEDGMENTS
The authors thank Dr Dong-Chul Han (Department of Internal Medicine, Soonchunhyang University, Seoul, Korea) for cell culture preparation. We thank Dr Dae Joong Kim, Dr Wooseong Huh and Dr Ha Young Oh (Samsung Medical Center, Sungkyunkwan University School of Medicine, Seoul, Korea) for their helpful advice on this study. We are also grateful to Young Ok Kim and Hyun Joong Kim for their technical assistance and their useful comments on the paper.
References
1. Nangaku M. Chronic hypoxia and tubulointerstitial injury: a final common pathway to end-stage renal failure. J Am Soc Nephrol. 2006. 17:17–25.


2. Fine LG, Bandyopadhay D, Norman JT. Is there a common mechanism for the progression of different types of renal diseases other than proteinuria? Towards the unifying theme of chronic hypoxia. Kidney Int Suppl. 2000. 75:S22–S26.


3. Norman JT, Orphanides C, Garcia P, Fine LG. Hypoxia-induced changes in extracellular matrix metabolism in renal cells. Exp Nephrol. 1999. 7:463–469.


4. Kim SB, Kang SA, Park JS, Lee JS, Hong CD. Effect of hypoxia on the extracellular matrix production of cultured rat mesangial cells. Nephron. 1996. 72:275–280.
5. Sahai A, Mei C, Pattison TA, Tannen RL. Chronic hypoxia induces proliferation of cultured mesangial cells: role of calcium and protein kinase C. Am J Physiol. 1997. 273:F954–F960.
6. Norman JT, Clark IM, Garcia PL. Hypoxia promotes fibrogenesis in human renal fibroblasts. Kidney Int. 2000. 58:2351–2366.


7. Manotham K, Tanaka T, Matsumoto M, Ohse T, Inagi R, Miyata T, Kurokawa K, Fujita T, Ingelfinger JR, Nangaku M. Transdifferentiation of cultured tubular cells induced by hypoxia. Kidney Int. 2004. 65:871–880.


8. Matsumoto M, Tanaka T, Yamamoto T, Noiri E, Miyata T, Inagi R, Fujita T, Nangaku M. Hypoperfusion of peritubular capillaries induces chronic hypoxia before progression of tubulointerstitial injury in a progressive model of rat glomerulonephritis. J Am Soc Nephrol. 2004. 15:1574–1581.


9. Choi YJ, Chakraborty S, Nguyen V, Nguyen C, Kim BK, Shim SI, Suki WN, Truong LD. Peritubular capillary loss is associated with chronic tubulointerstitial injury in human kidney: altered expression of vascular endothelial growth factor. Hum Pathol. 2000. 31:1491–1497.


10. Sahai A, Mei C, Schrier RW, Tannen RL. Mechanisms of chronic hypoxia-induced renal cell growth. Kidney Int. 1999. 56:1277–1281.


11. Blom IE, Goldschmeding R, Leask A. Gene regulation of connective growth factor: new targets for antifibrotic therapy? Matrix Biol. 2002. 21:473–482.
12. Chen Y, Blom IE, Sa S, Goldschmeding R, Abraham DJ, Leask A. CTGF expression in mesangial cells: involvement of SMADs, MAP kinase, and PKC. Kidney Int. 2002. 62:1149–1159.


13. Kondo S, Kubota S, Shimo T, Nishida T, Yosimichi G, Eguchi T, Sugahara T, Takigawa M. Connective tissue growth factor increased by hypoxia may initiate angiogenesis in collaboration with matrix metalloproteinases. Carcinogenesis. 2002. 23:769–776.


14. Higgins DF, Biju MP, Akai Y, Wutz A, Johnson RS, Haase VH. Hypoxic induction of CTGF is directly mediated by Hif-1. Am J Physiol Renal Physiol. 2004. 287:F1223–F1232.
15. Haverty TP, Kelly CJ, Hines WH, Amenta PS, Watanabe M, Harper RA, Kefalides NA, Neilson EG. Characterization of a renal tubular epithelial cell line which secretes theautologous target antigen of autoimmune experimental interstitial nephritis. J Cell Biol. 1988. 107:1359–1368.
16. Hayashida T, Poncelet AC, Hubchak SC, Schnaper HW. TGF-beta1 activates MAP kinase in human mesangial cells: a possible role in collagen expression. Kidney Int. 1999. 56:1710–1720.
17. Hartsough MT, Mulder KM. Transforming growth factor beta activation of p44mapk in proliferating cultures of epithelial cells. J Biol Chem. 1995. 270:7117–7124.
18. Ravanti L, Häkkinen L, Larjava H, Saarialho-Kere U, Foschi M, Han J, Kähäri VM. Transforming growth factor-beta induces collagenase-3 expression by human gingival fibroblasts via p38 mitogen-activated protein kinase. J Biol Chem. 1999. 274:37292–37300.
19. Kucich U, Rosenbloom JC, Herrick DJ, Abrams WR, Hamilton AD, Sebti SM, Rosenbloom J. Signaling events required for transforming growth factor-β stimulation of connective tissue growth factor expression by cultured human lung fibroblasts. Arch Biochem Biophys. 2001. 395:103–112.


20. Paradis V, Dargere D, Bonvoust F, Vidaud M, Segarini P, Bedossa P. Effects and regulation of connective tissue growth factor on hepatic stellate cells. Lab Invest. 2002. 82:767–774.


21. Zhang H, Akman HO, Smith EL, Zhao J, Murphy-Ullrich JE, Batuman OA. Cellular response to hypoxia involves signaling via Smad proteins. Blood. 2003. 101:2253–2260.


22. Maxwell P. HIF-1: an oxygen response system with special relevance to the kidney. J Am Soc Nephrol. 2003. 14:2712–2722.


24. Cummins EP, Comerford KM, Scholz C, Bruning U, Taylor CT. Hypoxic regulation of NF-kappaB signaling. Methods Enzymol. 2007. 435:479–492.
25. Minchenko A, Bauer T, Salceda S, Caro J. Hypoxic stimulation of vascular endothelial growth factor expression in vitro and in vivo. Lab Invest. 1994. 71:374–379.
26. Nakagawa T, Lan HY, Zhu HJ, Kang DH, Schreiner GF, Johnson RJ. Differential regulation of VEGF by TGF-β and hypoxia in rat proximal tubular cells. Am J Physiol Renal Physiol. 2004. 287:F658–F664.


27. Orphanides C, Fine LG, Norman JT. Hypoxia stimulates proximal tubular cell matrix production via a TGF-beta1-independent mechanism. Kidney Int. 1997. 52:637–647.
28. Gupta S, Clarkson MR, Duggan J, Brady HR. Connective tissue growth factor: potential role in glomerulosclerosis and tubulointerstitial fibrosis. Kidney Int. 2000. 58:1389–1399.


29. Xu J, Smock SL, Safadi FF, Rosenzweig AB, Odgren PR, Marks SC Jr, Owen TA, Popoff SN. Cloning the full-length cDNA for rat connective tissue growth factor: implications for skeletal development. J Cell Biochem. 2000. 77:103–115.

