Abstract
TRPV5 is believed to play an important role in the regulation of urinary calcium excretion. We assessed the effects of hydrochlorothiazide (HCTZ) on the expression of TRPV5, calbindin-D28K, and several sodium transporters in hypercalciuric rats. Sprague-Dawley rats were divided into 4 groups; control, HCTZ, high salt, and high salt with HCTZ group in experiment 1; control, HCTZ, high calcium (Ca), and high Ca with HCTZ group in experiment 2. To quantitate the expression of TRPV5, calbindin-D28K, and sodium transporters, western blotting was performed. In both experiments, HCTZ significantly decreased urinary calcium excretion. TRPV5 protein abundance decreased in all hypercalciuric rats, and restored by HCTZ in both high salt with HCTZ and high Ca with HCTZ group. Calbindin-D28K protein abundance increased in the high salt and high salt with HCTZ groups, but did not differ among groups in experiment 2. Protein abundance of NHE3 and NKCC2 decreased in all hypercalciuric rats, and were restored by HCTZ in only high Ca-induced hypercalciuric rats. In summary, protein abundance of TRPV5, NHE3, and NKCC2 decreased in all hypercalciuric rats. The hypocalciuric effect of HCTZ is associated with increased protein abundance of TRPV5 in high salt or calcium diet-induced hypercalciuric rats.
Calcium plays essential roles in neural excitation, muscle contraction, blood conjugation, bone formation, hormone secretion, cell adhesion, and enzyme activation. Blood concentration of calcium is tightly controlled within a narrow range by harmonious cooperation between three organs; kidney, bone, and intestine.
The kidneys contribute to calcium homeostasis by controlling calcium excretion. Only unbound calcium, which is 60% of total calcium, is filtered and most of the filtered calcium is reabsorbed in the proximal tubule (PT) and the thick ascending limb (TAL) passively via paracellular pathway driven by sodium and water reabsorption (1-3). Fine regulation of calcium excretion occurs in the distal nephron, where small fraction of filtered calcium is reabsorbed by active transcellular calcium transport. Transporters involved in this process include transient receptor potential vanilloid 5 (TRPV5) and transient receptor potential vanilloid 6 (TRPV6) on the luminal membrane, calbindin-D28K in the cytoplasm, and Na+-Ca2+ exchanger type 1 (NCX1) and plasma membrane Ca2+-ATP ase (PMCA) on the basolateral membrane (4). TRPV5 has been considered to play the most important role in active calcium reabsorption and its coexistence with calbindin-D28K, NCX1, and PMCA has been proved by immunohistochemistry (5).
Renal loss of calcium is one of the proposed mechanisms of idiopathic hypercalciuria. Idiopathic hypercalciuria is characterized by urinary calcium excretion of >0.1 mM/kg/day (>4 mg/kg/day) without a definite underlying cause (6, 7). Despite being named by Albright about 50 yr ago (8), the pathophysiology is still unclear and diagnosis remains exclusive. Proposed pathogenic mechanisms are increased intestinal calcium reabsorption, increased renal loss, and augmented bone resorption (9, 10).
Thiazide diuretics, which inhibit sodium-chloride cotransporter (NCC) in the distal convoluted tubule, have been adopted as a treatment of choice for idiopathic hypercalciuria due to their hypocalciuric effect (11). Although several reports demonstrated that thiazides increase bone mineral density and consequently reduce the risk of fracture (12-14), the mechanism of the hypocalciuric effect of thiazides and its effects on calcium transporters in the distal convoluted tubule are still controversial.
The purpose of this study was to elucidate mechanisms of the hypocalciuric effect of thiazides in high salt diet or high calcium diet-induced hypercalciuric rats. We analyzed the expression of TRPV5, calbindin-D28K, and sodium transporters of the PT and TAL in hypercalciuric rats and the changes of them after hydrochlorothiazide (HCTZ) administration.
Forty specific pathogen-free male Sprague-Dawley rats (6 weeks, 160-180 g; SLC, Shizuoka, Japan) were placed in separate metabolic cages 3 days before the beginning of the study. All rats were provided with fixed amount of finely ground regular rat chow (14.7 g/200 g of body weight per day) containing 0.3% (0.05 mM/g) NaCl and 1.3% (0.16 mM/g) CaCO3. The rats were allowed free access to drinking water containing 0.8% NaCl and 0.1% KCl. All studies were approved by the institutional board for animal research (IACUC).
Twenty rats were randomly allocated to the control (N1) group (NaCl 0.05 mM/g of chow, n=5), the HCTZ (T1) group (NaCl 0.05 mM/g of chow & HCTZ 7 mg/day, n=5), the high salt (S) group (NaCl 1.32 mM/g of chow, n=5), and the high salt with HCTZ (ST) group (NaCl 1.32 mM/g of chow & HCTZ 7 mg/day, n=5). Rats in the S and ST groups were provided with rat chow containing 8% (1.32 mM/g) NaCl (Sigma, St. Louis, MO, U.S.A.) of chow (14.7 g/200 g of body weight per day). Rats in the N1 and T1 groups were given the same amount of regular rat chow. Osmotic minipumps (model 2ML1, Alzet, Palo Alto, CA, U.S.A.) were implanted subcutaneously in the T1 and ST groups to deliver 7 mg/day HCTZ for 7 days. HCTZ was dissolved in 1.7% ethanolamine. And the osmotic minipumps containing vehicle only were implanted in the N1 and S groups. On day 7, all rats were sacrificed after bilateral nephrectomy and blood sampling under anesthesia using ethyl carbamate (33 g in 100 mL PBS, 0.5 cc/200 g body weight, intraperitoneal injection).
Twenty rats were randomly allocated to the control (N2) group (CaCO3 0.16 mM/g of chow, n=5), the HCTZ (T2) group (CaCO3 0.16 mM/g of chow & HCTZ 7 mg/day, n=5), the high Ca (calcium diet; C) group (CaCO3 0.74 mM/g of chow, n=5), and the high Ca with HCTZ (CT) group (CaCO3 0.74 mM/g of chow & HCTZ 7 mg/day, n=5). Rats in the C and CT groups were provided with rat chow containing 5% (0.74 mM/g) CaCO3 (Sigma) of chow (14.7 g/200 g of body weight per day). Rats in the N2 and T2 groups were given the same amount of regular rat chow. Osmotic minipumps (model 2ML1, Alzet) were implanted subcutaneously in the T2 and CT groups to deliver 7 mg/day HCTZ for 7 days. And the osmotic minipumps containing only vehicle were implanted in the N2 and C groups. On day 7, all rats were sacrificed after bilateral nephrectomy and blood sampling under anesthesia using ethyl carbamate.
In both experiments, body weight, water intake, and urine volume were measured daily. Urinary calcium was measured using Automated Electrolyte Analyzer EA07S (A&T, Yokohama-shi, Knagawa, Japan) and urinary creatinine was measured using Automatic Anaylzer 7170 (HITACHI, Ibaraki, Japan) in the first and last 24-hr urine. Calcium excretion was expressed as the calcium to creatinine ratio. Also, serum sodium and calcium levels were measured using Toshiba 200FR-1 (Toshiba, Tokyo, Japan) and hematocrit levels were measured using Automated Hematology Analyzer XE-2100 (Sysmex, Kobe, Japan).
The kidneys were dissected into small pieces and placed in a chilled isolation buffer containing 0.3 M sucrose, 10 mM triethanolamine (Sigma), 1 µg/mL leupeptin (Sigma), and 0.1 mg/mL phenylmethylsulfonylfluoride (Sigma) titrated to pH 7.6. Then the pieces were homogenized at 15,000 rpm with three stokes for 15 sec with a tissue homogenizer (Power Gun 125, Fisher Scientific, Pittsburgh, PA, U.S.A.). After homogenization, total protein concentration of the homogenate was measured by Bicinchoninic acid protein assay method (BCA Reagent Kit; Sigma) and diluted to 2.50 µg/mL using the isolation buffer solution. The samples were then stabilized by heating to 60℃ for 15 min after adding 1 vol 5×Laemmli sample buffer/4 vol sample.
Initially, "loading gels" were done on each sample set to calculate the exact loading amount that would guarantee equal loading on subsequent immunoblots. Ten micrograms of protein from each sample were loaded into each individual lane and electrophoresed on 12% polyacrylamide-SDS minigels by using Mini-PROTEIN III electrophoresis apparatus (Bio-Rad, Hercules, CA, U.S.A.) and then were stained with Coomassie blue dye (0.025% solution made in 4.5% methanol and 1% acetic acid; G-250, Bio-Rad). Selected bands from these gels were scanned with image analyzer (Vilber Lourmat, Marne la Vallée, France) to semiquantitatively determine density with LabWorks version 4.5 software (Ultra-Violet Products, Cambridge, U.K.) and relative amounts of protein loaded in each lane. Protein concentrations were corrected to reflect these measurements by repeating the process as described above.
For semiquantitative immunoblotting, the proteins electrophoresed on gels were transferred from unstained gels to nitrocellulose membrane (Bio-Rad) using the electroelution method. After being blocked with 5% skim milk in PBS-T (80 mM Na2HPO4, 20 mM NaH2PO4, 100 mM NaCl, and 0.1% Tween-20, pH 7.5) for 30 min, membranes were probed overnight at 4℃ with the respective primary antibodies. Affinity-purified immunoglobulin against TRPV5 (CHEMICON International, Temecula, CA, U.S.A.) and affinity-purified polyclonal antibodies for calbindin-D28K (ALPHA DIAGNOSTIC International, San Antonio, CA, U.S.A.) were used. The affinity-purified polyclonal antibodies against sodium-potassium-chloride cotransporter type 2 (NKCC2) and NCC were provided by Dr. HM Kwon (University of Maryland, Baltimore, MD, U.S.A.), and the antibodies against sodium-hydrogen exchanger 3 (NHE3) were provided by Dr. MA Knepper (NIH, Bethesda, MD, U.S.A.). Affinity-purified polyclonal antibodies against sodium-glucose cotransporter 1 (SGLT1) (CHEMICON International) were also used. For probing blots, all primary antibodies were diluted into a solution containing 150 mM NaCl, 50 mM sodium phosphate, 10 mg/dL sodium azide, 50 mg/dL Tween 20, and 0.1 g/dL bovine serum albumin (pH 7.5). Then, the membranes were cleaned with PBS-T and probed with secondary antibodies for 1 hr at room temperature. The secondary antibody was horseradish peroxidase-conjugated donkey anti-rabbit IgG (31458; Pierce, Rockford, IL) diluted to 1:3,000 except for calbindin-D28K. The secondary antibody for calbindin-D28K was horseradish peroxidase-conjugated rabbit anti-mouse IgG (31452; Pierce, Rockford, IL, U.S.A.) diluted to 1:5,000. Antibody-antigen reaction sites were viewed with enhanced chemiluminescence system (ECLTM RPN 2106; Amersham Pharmacia Biotech, Buckinghamshire, U.K.) before exposing to radiography films (Hyperfilm, Amersham Pharmacia Biotech, Little Chalfont, U.K.). Band density was measured by densitometry with Lab Works version 4.5 software (Ultra-Violet Products, Cambridge, U.K.) and calculated as a value relative to the average value of the control group.
All values are expressed as mean±standard deviation (SD). Band density values were normalized by dividing them with the average value of the control group. Thus the mean for the control group was defined as 100%. Group means of physiologic data were compared with ANOVA followed by Newman-Keuls post hoc analysis using GraphPad Prism version 4 (GraphPad Software, San Diego, CA, U.S.A.). Group means of protein abundance obtained from immunoblotting were compared with Mann-Whitney test using SPSS 12.0K. A p-value <0.05 was considered statistically significant.
There was no significant difference in body weight, hematocrit, and serum sodium concentration among groups (Table 1).
Urine Ca/Cr on day 0 was not significantly different among groups. On day 7, urine Ca/Cr was significantly increased in the high salt group, and decreased in the high salt with HCTZ group when compared to the high salt group (control vs. HCTZ vs. high salt vs. high salt with HCTZ, 0.39±0.15 vs. 0.19±0.07 vs. 1.52±0.51 vs. 0.62±0.19; p<0.05). Urine Ca/Cr in the high salt with HCTZ group was 41% of the level in the high salt group (Fig. 1). Urine Ca/Cr is summarized in Table 2.
TRPV5 protein abundance was significantly increased in the HCTZ group, decreased in the high salt group, and restored in the high salt with HCTZ group when compared to the high salt group (band density [%]; control vs. HCTZ vs. high salt vs. high salt with HCTZ, 100±18.02 vs. 184.27±41.49 vs. 69.07±15.18 vs. 78.29±33.57; Fig. 2). Calbindin-D28K protein abundance was significantly increased in the high salt and the high salt with HCTZ groups (band density [%]; control vs. HCTZ vs. high salt vs. high salt with HCTZ, 100±42.33 vs. 94.10±12.79 vs. 222.63±12.24 vs. 258.44±92.26; Fig. 3). Protein abundance of NHE3, SGLT1, NKCC2, and NCC increased in the HCTZ group, but protein abundance of NHE3 and NKCC2 decreased in the high salt group (band density: control vs. HCTZ vs. high salt vs. high salt with HCTZ, NHE3 [%]; 100±31.83 vs. 126.59±21.68 vs. 5.36±1.13 vs. 1.33±0.69, SGLT1 [%]; 100±22.23 vs. 182.45±22.66 vs. 146.53±5.26 vs. 125.61±12.67, NKCC2 [%]; 100±11.14 vs. 139.48±8.60 vs. 61.15±19.89 vs. 54.56±13.73, NCC [%]; 100±4.74 vs. 133.11±17.22 vs. 98.61±9.40 vs. 66.60±14.15; Fig. 4).
Changes in body weight, hematocrit, and serum sodium concentration did not differ significantly among groups (Table 3).
Urine Ca/Cr on day 0 was not significantly different among groups. On day 7, urine Ca/Cr was significantly increased in the high Ca group (Fig. 5). In the high Ca with HCTZ group, urine Ca/Cr was decreased to about 47% levels of high Ca group (control vs. HCTZ vs. high Ca vs. high Ca with HCTZ, 0.40±0.16 vs. 0.19±0.08 vs. 2.49±0.51 vs. 1.17±0.05; p<0.05). Urine Ca/Cr is summarized in Table 4.
Immunoblotting for TRPV5 revealed significantly decreased band density in the high Ca group and restored band density in the high Ca with HCTZ group (band density [%]; control vs. HCTZ vs. high Ca vs. high Ca with HCTZ, 100±18.92 vs. 168.04±29.83 vs. 60.06±9.31 vs. 123.77±23.90; Fig. 6). Protein abundance of calbindin-D28K did not differ significantly among groups (band density [%]; control vs. HCTZ vs. high Ca vs. high Ca with HCTZ, 100±22.18 vs. 102.68±11.42 vs. 101.66±24.24 vs. 94.54±29.38; Fig. 7). Protein abundance of NHE3, SGLT1, and NKCC2 was significantly decreased in the high Ca group and restored in the high Ca with HCTZ group (band density: control vs. HCTZ vs. high Ca vs. high Ca with HCTZ, NHE3 [%]; 100±11.91 vs. 116.20±11.11 vs. 8.63±4.26 vs. 45.77±26.29, SGLT1 [%]; 100±45.47 vs. 240.07±11.08 vs. 51.73±8.05 vs. 95.60±25.47, NKCC2 [%]; 100±8.66 vs. 123.08±1.56 vs. 66.05±4.72 vs. 80.16±17.66, NCC [%]; 100±26.18 vs. 161.07±30.44 vs. 62.49±28.93 vs. 67.14±9.93; Fig. 8).
To reveal the mechanisms of hypocalciuric effect of thiazide, we analyzed and compared changes of TRPV5 and major sodium transporters before and after HCTZ administration in both normal and hypercalciuric conditions induced by diet intervention. Regarding hypocalciuric effect of thiazide, there has been no report examining both major calcium and sodium transporters.
Protein abundance of TRPV5, NHE3, and NKCC2 was significantly decreased in all hypercalciuric rats. Our results imply that hypercalciuria may be caused by dysregulation of several stages of calcium transportation in the kidneys (15). Approximately 70% of calcium reabsorption occurs in the proximal tubule, predominantly through paracellular pathways with salt and water carrying calcium from the lumen to the interstitium by solvent drag. Approximately 20% of filtered calcium is reabsorbed in the TAL of the loop of Henle via both paracellular and transcellular processes. In the TAL, the driving force for paracellular transport of calcium is provided by a lumen-positive voltage generated by the NKCC2. The remaining approximately 10% of filtered calcium is reabsorbed in the distal convoluted tubule and connecting tubule; reabsorption in these segments is predominantly mediated by active transcellular transport under hormonal regulation (3, 16-18).
TRPV5 is confined to the apical domain of the epithelial cells lining the distal part of the distal convoluted tubule and the connecting tubule (5, 19). Other proteins involved in calcium transport such as calbindin-D28K, Na+-Ca2+ exchanger type 1, and plasma membrane Ca2+-ATPase 1b are also located in these segments, but they are distributed in the cytoplasm or on the basolateral membrane (5). Although TRPV6 is also expressed in these segments, it is concentrated in the small intestine and the affinity to calcium is 1/100 of that of TRPV5 (4). Therefore, TRPV5 can be expected to have a major role in active calcium reabsorption in the distal convoluted tubule.
Our results coincide with the basic phenomena mentioned above showing significant decrease of TRPV5, NHE3, and NKCC2 protein abundance in all hypercalciuric rats. Although protein abundance of NHE3 and NKCC2 was decreased in all hypercalciuric rats regardless of experimental protocols, there seemed to be different underlying mechanisms. In experiment 1, abundance of sodium and chloride may have resulted in significant decrease of NHE3 and NKCC2. On the other hand, in experiment 2, abundant carbon dioxide released from CaCO3 may have induced alkalemic condition, and consequently facilitated bicarbonate excretion by down-regulation of NHE3 and NKCC2 because the normovolemic condition was maintained by providing electrolyte solution. It is already well known that bicarbonate excretion will rise only if the stimulus to sodium and chloride retention is removed by the restoration of normovolemia, and acid-base balance has influence on NHE3 and NKCC2 (2). However, some reported contradictory results about the changes of NHE3 in dietary NaCl intake and of NKCC2 in saline loading (20, 21). Discrepancy between our data and their results seemed to be caused from different experiment protocols, specifically, NaCl amount. In our experiment 1, we loaded much more salt than previous studies.
Changes of calbindin-D28K protein abundance differed significantly depending on the protocols used to induce hypercalciuria. Calbindin-D28K protein abundance did not change in high calcium diet-induced hypercalciuric rats, but significantly increased in high salt diet-induced hypercalciuric rats suggesting that transporters on the luminal side may play a more substantial role in the regulation of calcium excretion and calbindin-D28K may not be a critical determining factor of hypercalciuria. One previous report using calbindin-D28K knockout mice also demonstrated that calbindin-D28K did not play an important role in the development of hypercalciuria (22). In that study, calbindin-D28K knockout mice did not show hypercalciuria, whereas TRPV5 knockout mice and TRPV5/calbindin-D28K double knockout mice showed similar degrees of hypercalciuria. Our results also imply that major transporters participating in both active and passive calcium reabsorption are associated with the development of hypercalciuria. Further studies are needed to elucidate the functional correlation between TRPV5 and calbindin-D28K after HCTZ treatment in hypercalciuria.
Although the degree of hypercalciuria induced by high salt diet was less severe than that by high calcium diet, high salt diet itself caused significant hypercalciuria. The clinically harmful effect of high salt diet on urinary calcium excretion was already proposed in the 1960's (23, 24). Borghi et al. (25) reported that animal protein and salt increased calcium excretion. Our results support the previous clinical observation providing a possible physiologic explanation.
In the HCTZ group, protein abundance of NHE3, SGLT1, NKCC2, and NCC was increased. Upregulation of NCC protein abundance after chronic HCTZ treatment was previously reported (26). Protein abundance of TRPV5 was significantly increased in the HCTZ group. Changes in protein abundance of TRPV5 and major sodium transporters of the PT and TAL coincide with the hypocalciuric effect of HCTZ, and imply that both TRPV5 and sodium transporters collaborate reducing calcium excretion in rats fed with normal diet after HCTZ administration.
HCTZ resulted in significant reduction of urinary calcium excretion in all hypercalciuric rats, and the degree of hypocalciuric effect was similar regardless the protocols inducing hypercalciuria; in the high salt with HCTZ group, urine Ca/Cr decreased to about 41% of the high salt group; in the high Ca with HCTZ group, urine Ca/Cr decreased to about 47% of the high Ca group.
Several distinct hypotheses have been proposed concerning the hypocalciuric effect of HCTZ. Nijenhuis et al. reported that HCTZ-induced hypocalciuria is accompanied by a decrease in TRPV5, Na+-Ca2+ exchanger, and calbindin-D28K in rat kidneys and that extracellular volume contraction is a critical determinant of HCTZ-induced hypocalciuria (27). Lee et al. proposed that HCTZ induces hypocalciuria through different mechanisms depending on volume status (28). Nijenhuis et al. suggested that enhanced passive calcium reabsorption is more important for HCTZ-induced hypocalciuria than active calcium reabsorption (29). In this study, the rats were provided with drinking water containing 0.8% NaCl and 0.1% KCl to prevent volume depletion, and measured data such as weight gain, hematocrit, and serum sodium concentration showed no difference among groups, reflecting a comparable volume status.
Protein abundance of TRPV5 was increased by HCTZ in all hypercalciuric rats fed with high salt or high calcium diet. However, protein abundance of NHE3, SGLT1, and NKCC2 was increased by HCTZ in the high Ca with HCTZ group, but was not affected by HCTZ in the high salt with HCTZ group. Despite the different effect of HCTZ on NHE3, SGLT1, and NKCC2 in hypercalciuric rats, HCTZ showed a similar hypocalciuric effect in both experiments; about 50% reduction of calcium excretion by HCTZ. This suggests that TRPV5 has a more crucial function in the regulation of calcium excretion in hypercalciuric conditions. Our results are compatible with the previous report by Hoenderop et al. (30) demonstrating the essential role of TRPV5 in calcium homeostasis using TRPV5-null mice, and the report by Gkika et al. (22) showing that the gatekeeper function of TRPV5 is the rate-limiting step in active calcium reabsorption using single- and doubleknockout mice lacking TRPV5 and calbindin-D28K.
In conclusion, decreased protein abundance of TRPV5, NHE3, and NKCC2 is associated with the development of hypercalciuria, and changes in protein abundance of TRPV5 may be related to the HCTZ-induced hypocalciuric effect in both normal and hypercalciuric conditions. Our data suggest that TRPV5 is a critical determinant of the hypocalciuric effect of chronic HCTZ treatment.
Figures and Tables
Fig. 1
Urine Ca/Cr ratio on day 7 in high salt diet-induced hypercalciuric rats. Urine Ca/Cr was significantly increased in the high salt group, and decreased in the high salt with HCTZ group when compared to the high salt group.
*p<0.05 when compared to the control group; †p<0.05 when compared to the high salt group.
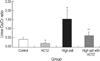
Fig. 2
TRPV5 expression in high salt diet-induced hypercalciuric rats. TRPV5 protein abundance was significantly increased in the HCTZ group, decreased in the high salt group, and increased in the high salt with HCTZ group when compared to the high salt group.
*p<0.05 when compared to the control group; †p<0.05 when compared to the high salt group.
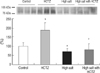
Fig. 3
Calbindin-D28K expression in high salt diet-induced hypercalciuric rats. Calbindin-D28K protein abundance was significantly increased in both high salt and high salt with HCTZ groups.
*p<0.05 when compared to the control group.
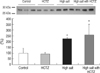
Fig. 4
Protein abundance of sodium transporters in high salt diet-induced hypercalciuric rats. Protein abundance of NHE3 and NKCC2 was increased in the HCTZ group, but decreased in the high salt group. NCC protein abundance increased in the HCTZ group, but was not changed in the high salt group. SGLT1 protein abundance increased in both HCTZ group and high salt group.
*p<0.05 when compared to the control group.
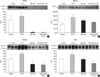
Fig. 5
Urine Ca/Cr ratio on day 7 in high calcium diet-induced hypercalciuric rats. Urine Ca/Cr was significantly increased in the high Ca group. In the high Ca with HCTZ group, urine Ca/Cr was decreased to about 47% of the high Ca group.
*p<0.05 when compared to the control group; †p<0.05 when compared to the high Ca group.
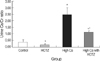
Fig. 6
TRPV5 expression in high calcium diet-induced hypercalciuric rats. TRPV5 protein abundance was significantly decreased in the high Ca group, but restored in the high Ca with HCTZ group.
*p<0.05 when compared to the control group; †p<0.05 when compared to the high Ca group.
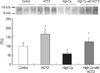
Fig. 7
Calbindin-D28K expression in high calcium diet-induced hypercalciuric rats. Protein abundance of calbindin-D28K was not different among groups.
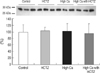
Fig. 8
Protein abundance of sodium transporters in high calcium diet-induced hypercalciuric rats. Protein abundance of NHE3, SGLT1, NKCC2, and NCC was decreased in the high Ca group. Protein abundance of NHE3, SGLT1, and NKCC2 was increased by HCTZ in the high Ca with HCTZ group when compared to the high Ca group.
*p<0.05 when compared to the control group; †p<0.05 when compared to the high Ca group.
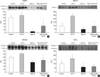
Table 1
Body weight gain, urine volume, hematocrit, and serum sodium concentration in high salt diet-induced hypercalciuric rats (n=5 in each group)

ACKNOWLEDGMENTS
We would like to thank Dr. HM Kwon and Dr. Mark A. Knepper for providing the antibodies for this study. We also thank MK Park for technical assistance.
References
2. Rose Burton David, Post Theodore W.. Proximal tubule: renal physiology. Clinical physiology of acid-base and electrolyte disorders. 2001. New York: McGraw-Hill;71–103.
3. Hoenderop JG, Nilius B, Bindels RJ. Molecular mechanism of active ca2+ reabsorption in the distal nephron. Annu Rev Physiol. 2002. 64:529–549.
4. Hoenderop JG, Nilius B, Bindels RJ. Epithelial calcium channels: from identification to function and regulation. Pflugers Arch. 2003. 446:304–308.


5. Hoenderop JG, Hartog A, Stuiver M, Doucet A, Willems PH, Bindels RJ. Localization of the epithelial ca (2+) channel in rabbit kidney and intestine. J Am Soc Nephrol. 2000. 11:1171–1178.
6. Bataille P, Fardellone P, Ghazali A, Cayrolle G, Hottelart C, Achard JM, Fournier A. Pathophysiology and treatment of idiopathic hypercalciuria. Curr Opin Rheumatol. 1998. 10:373–388.


8. Albright F HP, Benedict PH, Forebes AP. Idiopathic hypercalciuria: a preliminary report. Proc R Soc Med. 1953. 46:1077–1081.
9. Backman U, Danielson BG, Johansson G, Ljunghall S, Wikstrom B. Incidence and clinical importance of renal tubular defects in recurrent renal stone formers. Nephron. 1980. 25:96–101.


10. Martinez ME, Villa E, Vazquez Martul M, Sanchez-Cabezudo MJ, Sanchez JA, Villa JR. Influence of calcium intake on calcitriol levels in idiopathic hypercalciuria in children. Nephron. 1993. 65:36–39.


11. Costanzo LS, Weiner IM. On the hypocalciuric action of chlorothiazide. J Clin Invest. 1974. 54:628–637.


12. Ray WA, Griffin MR, Downey W, Melton LJ 3rd. Long-term use of thiazide diuretics and risk of hip fracture. Lancet. 1989. 1:687–690.


13. LaCroix AZ, Wienpahl J, White LR, Wallace RB, Scherr PA, George LK, Cornoni-Huntley J, Ostfeld AM. Thiazide diuretic agents and the incidence of hip fracture. N Engl J Med. 1990. 322:286–290.


14. Reid IR, Ames RW, Orr-Walker BJ, Clearwater JM, Horne AM, Evans MC, Murray MA, McNeil AR, Gamble GD. Hydrochlorothiazide reduces loss of cortical bone in normal postmenopausal women: a randomized controlled trial. Am J Med. 2000. 109:362–370.


15. Frick KK, Bushinsky DA. Molecular mechanisms of primary hypercalciuria. J Am Soc Nephrol. 2003. 14:1082–1095.


16. Bushinsky DA. Greenberg , editor. Disorders of calcium and phosphorus homeostasis. Primer on kidney diseases. 2001. San Diego: academic Press;107–115.
17. Scheinman SJ, Guay-Woodford LM, Thakker RV, Warnock DG. Genetic disorders of renal electrolyte transport. N Engl J Med. 1999. 340:1177–1187.


18. Reilly RF, Ellison DH. Mammalian distal tubule: physiology, pathophysiology, and molecular anatomy. Physiol Rev. 2000. 80:277–313.


19. Loffing J, Loffing-Cueni D, Valderrabano V, Klausli L, Hebert SC, Rossier BC, Hoenderop JG, Bindels RJ, Kaissling B. Distribution of transcellular calcium and sodium transport pathways along mouse distal nephron. Am J Physiol Renal Physiol. 2001. 281:F1021–F1027.
20. Eladari D, Leviel F, Pezy F, Paillard M, Chambrey R. Rat proximal nhe3 adapts to chronic acid-base disorders but not to chronic changes in dietary nacl intake. Am J Physiol Renal Physiol. 2002. 282:F835–F843.
21. Ecelbarger CA, Terris J, Hoyer JR, Nielsen S, Wade JB, Knepper MA. Localization and regulation of the rat renal na(+)-k(+)-2cl-cotransporter, bsc-1. Am J Physiol. 1996. 271:F619–F628.
22. Gkika D, Hsu YJ, van der Kemp AW, Christakos S, Bindels RJ, Hoenderop JG. Critical role of the epithelial ca2+ channel trpv5 in active ca2+ reabsorption as revealed by trpv5/calbindin-d28k knockout mice. J Am Soc Nephrol. 2006. 17:3020–3027.


23. Kleeman CR, Bohannan J, Bernstein D, Ling S, Maxwell MH. Effect of variations in sodium intake on calcium excretion in normal humans. Proc Soc Exp Biol Med. 1964. 115:29–32.


24. Phillips MJ, Cooke JN. Relation between urinary calcium and sodium in patients with idiopathic hypercalciuria. Lancet. 1967. 1:1354–1357.


25. Borghi L, Schianchi T, Meschi T, Guerra A, Allegri F, Maggiore U, Novarini A. Comparison of two diets for the prevention of recurrent stones in idiopathic hypercalciuria. N Engl J Med. 2002. 346:77–84.


26. Na KY, Oh YK, Han JS, Joo KW, Lee JS, Earm JH, Knepper MA, Kim GH. Upregulation of na+ transporter abundances in response to chronic thiazide or loop diuretic treatment in rats. Am J Physiol Renal Physiol. 2003. 284:F133–F143.
27. Nijenhuis T, Hoenderop JG, Loffing J, van der Kemp AW, van Os CH, Bindels RJ. Thiazide-induced hypocalciuria is accompanied by a decreased expression of ca2+ transport proteins in kidney. Kidney Int. 2003. 64:555–564.


28. Lee CT, Shang S, Lai LW, Yong KC, Lien YH. Effect of thiazide on renal gene expression of apical calcium channels and calbindins. Am J Physiol Renal Physiol. 2004. 287:F1164–F1170.

