Abstract
Figures and Tables
![]() | Fig. 1Chromosomal losses detected in the gastric, colonic, mammary, and nasopharyngeal cancers (25 cases for each cancer type). (A) Individual chromosomal losses and (B) the number of chromosomal losses were evaluated by PCR-based analysis using 40 microsatellite markers on chromosomes 3p, 4p, 5q, 8p, 9p, 13q, 17p, and 18q. |
![]() | Fig. 2Methylation changes in the transitional-CpG sites of the 15 selected genes examined in four cancer types. The methylation status of the transitional-CpG sites was estimated using a semiquantitative methylation-specific PCR method. The methylation changes were scored based on the difference in the level of methylation between the normal and tumor tissues. The frequency of methylation changes in each transitional area is indicated as a percentage in 25 cancer cases. The level of chromosomal losses was evaluated by PCR-based loss-of-heterozygosity analysis. The cancer tissues were grouped into high-level chromosomal losses (H) four or more chromosomes and low-level chromosomal losses (L) involving less than four chromosomes. |
![]() | Fig. 3Comparison of the transitional-CpG methylation changes between the different cancer types (A) and between cancers with high-level (H) and low-level (L) chromosomal losses (B). The criteria for the level of chromosomal losses are described in the legend of Fig. 2. The frequency of the methylation changes in the six non-island CpG sites and nine CpG-island margins are indicated as a percentage in 25 cancer cases. p values were calculated for the differences in the frequency of methylation changes between four cancer types by a chi-square test. |
![]() | Fig. 4Methylation profiles of 15 transitional CpG sites examined in 11 tissue types. The estimation of CpG methylation obtained using semiquantitative methylation-specific PCR were divided into five levels (○, 0-20%; ⊘, 21-40%; ⊗, 41-60%; ⊛, 61-80%; ●, 81-100%). Differences in the mean level of methylation between the most highly methylated and least methylated tissue types are indicated by closed bars. Somatic tissues were classified into three germ-layer lineages, endoderm (EN), mesoderm (ME), and ectoderm (EC). |
![]() | Fig. 5Transcript populations of the embryonic stem cells, placenta, and somatic tissues in the SAGE libraries. (A) The number of the active genes and the number of tags expressed per active gene were calculated separately according to the presence or absence of CpG islands. The mean values of two to eight tissues are indicated for each tissue type. N, normal; C, cancer. All information about the SAGE data is listed in Supplementary Table 4. (B) Transcripts of the CpG-island-negative genes close to the L1 and Alu retroelements. The genes lacking the CpG islands were grouped according to the type of retroelements round the transcription start sites. The relative proportion of the gene-group transcripts in the total transcript population was calculated for each normal and cancer tissue. The transcript data of the nasopharynx was obtained from the EST libraries. All information regarding individual tissues is listed in Supplementary Table 4. |
Table 1
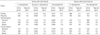
Table 2

Using the SAGE data in silico, the number of expressed tags of individual genes was compared between the embryo (embryonic cell line of passage 16 and the first trimester placenta) and somatic tissues. Total genes were classified according to the presence or absence of CpG islands and the type of the nearby retroelements. Pearson's correlation coefficients of p<0.05 are marked in bold. R values ≥0.5 are indicated by italics.
Table 3

The methylation of the CpG-island margins were divided into four levels (+, ++, +++, and ++++) according to the genome-wide DNA methylation of the embryo (27, 28) and the placenta (29) and the CpG-island margin methylation estimated in somatic tissues (Fig. 4). The number of active genes and the transcript number per active gene were divided into four or five levels according to the ranks observed in the embryo and somatic tissues (Fig. 5). Hypomethylation changes in cancer tissues are detailed in Fig. 3. NE, not examined.
References




















