Abstract
This study was conducted to investigate the metabolic changes in the motor and motor association cortices following axonal injury in the internal capsule that was caused by deep intracerebral hematoma. Using proton magnetic resonance spectroscopy (1H MRS), the authors studied the primary motor cortices (M-1) and sup-plementary motor areas (SMA) of 9 hemiparetic patients with documentable hemi-paresis of varying severity, and we studied 10 normal volunteers as controls. To measure the M-1 and SMA biochemical changes, 4 separate single volumes of inter-est(VOIs) were located bilaterally in the affected and unaffected hemisphere (AH and UH). 1H MRS provided a neuronal and axonal viability index by measuring levels of N-acetylaspartate (NAA) and creatine/phosphocreatine (Cr). The M-1/SMA NAA/Cr ratios of the AH and UH in patients, and the AH and normal volunteers were com-pared. The NAA/Cr ratios of the M-1 and SMA in AH, and the SMA in UH were sig-nificantly lower than those of normal volunteers. These 1H MRS findings indicate that axonal injury in the descending motor pathway at the level of internal capsule could induce metabolic changes in the higher centers of the motor pathway.
Proton magnetic resonance spectroscopy (1H MRS) is a noninvasive technique that allows us to provide an in vivo assessment of brain tissue composition and this gives us an insight into the metabolic processes of neurologic disorders (1, 2). This technique's ability to obtain good spatial localization and serial measurements with high resolution makes in vivo 1H MRS an ideal investigative tool of brain function, with respect to biochemical interactions (3). The application of this technique creates the possibility of observing major brain metabolites that contributes to the major resonances seen in 1H MR spectra of the brain, such as N-acetylaspartate (NAA), creatine/phosphocreatine (Cr), choline-containing compounds (Cho), and myo-inositiols (Ins) (1-3). Using current localization techniques, 1H MRS spectra can be obtained from regions of 1-10 µL within the timescale of a clinical examination, and this allows for the definition of spectral changes specific to the motor cortex (3).
Early studies of 1H MRS in stroke have mostly investigated ischemic stroke, and they have shown an increased lactate and decreased NAA within the stroke lesion (4-6). However, attempts to determine whether the magnitude of neuronal damage, as measured by NAA loss, correlates with the disability and impairment in ischemic stroke patients have brought forth inconsistent results (7-10). There has been no previous 1H MRS study that has investigated the metabolic changes in the higher motor cortex following intracerebral hematoma in hemiparetic humans. The aim of this study was to evaluate the local metabolic changes for the primary motor cortex (M-1) and supplementary motor area (SMA) in the affected hemisphere (AH) and unaffected hemisphere (UH), according to their axonal injuries at the level of the internal capsule.
The M-1/SMA of the AH and the UH were studied using 1H MRS on 9 patients (4 men and 5 women; mean age 54 yr, range 30-70 yr, right-handed) with documentable hemiparesis of varying severity. The patients' clinical details are summarized in Table 1. In all cases, the hemiparesis was caused by deep intracerebral hematoma in the putamen and the thalamus because these locations are directly adjacent to the internal capsule, and an intracerebral hematoma in these areas mostly brings about hemiparesis as a neurologic sequele. Neurosurgical inpatients and outpatients were recruited for the study. The 1H MRS study was performed on alert patients with definite hemiparesis of the extremities contralateral to the AH. To control the possible 1H MRS spectral change, we excluded those patients that had undergone any surgical intervention or if they had a major systemic illness, such as uremia.
The results were compared with 1H MRS studies performed on 10 normal control volunteers (five men and five women; mean age 41 yr, range 28-59 yr, all right-handed), and they were screened to exclude those people with prior systemic disease or head injury. The Institutional Review Board approved the MR study proctocol and the investigation was conducted in full compliance with accepted standards for research involving humans. An informed consent was obtained from all participants or their legal guardians. The mean duration of the study was approximately 1 yr, with a range of 7 days to 1 yr after development of intracerebral hematoma.
In vivo 1H MRS studies were performed on a 1.5 T MRI/MRS system (GE Signa Advantage, version 4.8; GE Medical Systems, Milwaukee, WI, U.S.A.) using the stimulated echo acquisition mode (STEAM) sequence after water suppression with chemical-shift selective (CHESS) RF pulse and dephasing gradients (11, 12). A standard head coil was used for the routine neurological and research imaging sequences, with image-guided single-voxel spectroscopic acquisition. A 6.4 mL volume in the cortex was selected using the T2-weighted MR images (90 msec TE, 2,500 msec TR). For examining the M-1 cortex with the single voxel technique, a cubic volumes of interest (VOI) of 18×18×20 mm was placed in the medial precentral gyrus (Broadman area 4) anterior to the central sulcus in both hemispheres (AH and UH), and for the SMA, the same-sized VOI was placed in the medial side of the superior frontal gyrus (Broadman area 6) just anterior to the M-1 cortex VOI, as is shown in Fig. 1. The voxel location was carefully chosen by two investigators to ensure the exact anatomical location of M-1/SMA for each patient.
The spectral parameters used were: 20 msec TE, 2,000 msec TR, 128 averages, 2,500 Hz spectral width, and 2,048 data points. The shim procedure was used for optimizing the magnetic field homogeneity over the entire volume of interest that was detected by the receiver coil and focused on the water signal. After an autoprescan, the typical line width (full length at half maximum; FWHM) was usually 2 to 4 Hz, and the raw data was processed using a SAGE data analysis package (GE Medical Systems). The post-processing was done using zerofilling to 4K, and apodization filtering was used to improve either SNR or resolution and/or to reduce the truncation artifacts. Fourier transformation, zero order phase correction, and phase absorption spectra were performed directly along with baseline corrections or resolution enhancement. The peak areas were obtained from the spectra using the Marquardt algorithm to fit a Lorentzian-Gaussian peak type (13). Proton peak assignments of the major in vivo 1H MRS observable metabolites were NAA at 2.00 ppm; Cr at 3.00 pm; Cho at 3.20 ppm; sum (Glx) of Glu and GABA at 2.35, and 2.25 ppm; and Ins at 3.50 pm (14). After the data was processed by our selected personal "blinded" to the patient's histories and conditions, the results were expressed as means±SD of the NAA/Cr ratio and the Cho/Cr ratios.
The metabolite ratios in the AH and UH and the controls' values were compared for significant differences using the paired t-test assuming equal variance and independent sample t-test. Probability values of less than 0.05 were considered significant, and values less than 0.01 were considered highly significant.
The results are summarized in Table 2. The NAA/Cr ratios of M-1 in the AH and UH were 1.08±0.12, 1.50±0.17, respectively. There were significant differences between the AH and UH in the NAA/Cr ratios of M-1 (p<0.05). The NAA/Cr ratios of M-1 measured in the AH and controls were 1.08±0.12, 1.37±0.12, respectively. There was also significant differences between the AH and controls in the NAA/Cr ratios of M-1 (p=0.01). However, the NAA/Cr ratios of M-1 measured in the UH showed no differences when compared with the ratios of normal volunteers (p=0.115). These results showed that the NAA/Cr ratios of the AH were significantly decreased when compared with those of the UH and normal volunteers and therefore, the metabolic changes had occurred in the high cortical region ipsilateral to the injured hemispheres. However, the NAA/Cr ratios of M1 in the AH showed no significant correlation with the severity of arm weakness, leg weakness, or the duration of hemiparesis.
The NAA/Cr ratios of the SMA measured in the AH and UH were 1.18±0.15, 1.18±0.27, respectively. There was no difference between the AH and UH in the NAA/Cr ratios of the SMA (p=0.980). However, when compared with the SMA of the controls, the NAA/Cr ratios of SMA in the AH and UH showed significant differences (p<0.05).
In terms of Cho/Cr ratios, no significant differences were found between the following; the M1 cortices of the AH and UH (p=0.316), the SMAs of the AH and UH (p=0.561), the M-1 cortex and SMA of the AH (p=0.745), and the M-1 cortex and SMA of the UH (p=0.289). No significant differences were found when comparing the Cho/Cr ratios of each cortex with those of the normal volunteers. The lactate resonance, which we expected to see at 1.3 ppm, was not observed in any of these cases. The NAA/Cr and Cho/Cr ratios of the M1 and SMA in the control group did not show any difference between right and left hemispheres (Fig. 2).
Hemiparesis is the most commonly experienced deficit after stroke, and it acutely affects more than 80% of subjects and chronically affects greater than 40% of the subjects (15). Patients show a wide range for the degree of recovery, and the restorative processes that occur in the brain after stroke remain incompletely understood. This work focused on the neurochemical profile of the motor cortex and the supplementary motor area in relation with pyramidal tract injury caused by deep intracerebral hematoma.
1H MRS provides chemical information on the local cellular metabolism by using the metabolic ratios of neurochemicals detected in the spectra (11, 12). One of the most important contributions of 1H MRS to clinical neuroscience is its ability to quantify neuronal loss and to demonstrate reversible neuronal damage (1, 2). 1H MRS is a useful research tool for elucidating the pathophysiology underlying certain diseases, including brain tumors, head trauma, cerebrovascular, neurodegenerative, and metabolic brain diseases (1).
1H MRS has been used in attempting better qualification of the brain damage caused by stroke. 1H MRS allows the in vivo measurement of N-acetyl-containing compounds, creatine, choline, and lactate. The majority of the N-acetyl signal comes from NAA, which is present in high concentrations in the brain (16). The function of NAA is unclear, although suggestions have included the initiation of protein synthesis, neurotransmission, and the deactivation of glutamate. NAA is of particular interest in studies of the brain because it is located almost exclusively in the neurons of adults (17). Initially, it was suggested that decrease in NAA represented neuronal loss (18); however, a reversible decrease in NAA has recently been shown to occur for acute lesions in multiple sclerosis and in stroke-like episodes of MELAS (19). Further, NAA synthesis in vitro has been shown to occur in an energy-dependent manner, and this is reduced by mitochondrial inhibitors (20). Therefore, decrease in the NAA resonance peak in vivo must be interpreted as an index of neuronal or axonal injury rather than as a marker of neuronal or axonal loss (21). Decrease in NAA have been shown to correlate with disability in multiple sclerosis (22), suggesting that axonal injury is responsible for the chronic functional impairment in this disease.
Early studies of 1H MRS for stroke have shown increased lactate and decreased NAA within the stroke lesion (4-6). Subsequent attempts have been made to determine whether the magnitude of neuronal damage, as measured by the NAA loss from the infarcted region, correlated with the disability and impairment in stroke patients. Ford et al. (8) found that the patients who made the most complete recoveries were those in whom NAA levels were relatively well preserved. In contrast, Gideon et al. (9) found no clear relationship between the level of NAA and the patient's clinical outcome. Graham et al. (10) found that NAA reduction correlated with the Barthel index score at discharge. Federico et al. (7) found that the NAA loss measured during the first week after stroke in patients with a speech or motor deficit correlated with the Scandinavian Stroke Scale and the Barthel index at 6 months.
However, some authors have criticized these findings because it remained unclear whether NAA loss was a better prognostic indicator than other measures, such as infarct volume as measured on imaging (21). Pendlebury et al. (21) has pointed out that the above-mentioned studies regarding 1H MRS and outcome after stroke measured the NAA loss from the center of infarcted region, and thus these NAA losses were not representative of the total neuronal injury. Moreover, the NAA loss was not measured in a specific brain region directly relevant to the chosen outcome measures. To examine the relationship between NAA loss after stroke and motor outcome, the researchers studied NAA levels in the posterior limb of the internal capsule, which contains the descending motor pathways for patients with motor deficits secondary to a cortical, subcortical, or capsular stroke. In that study, they found that mean internal capsule NAA was significantly lower in the patient group and axonal injury in the descending motor pathways at the level of internal capsule correlated with motor deficit for patients after stroke. They suggested a possible anterograde axonal injury might have an important role for this NAA loss in strokes involving the internal capsule and the motor and subcortex areas that contains the motor pathway (21).
Although there have been many 1H MRS studies dealing motor deficit in ischemic stroke, 1H MRS studies investigating the motor system in hemorrhagic stroke, especially in the intracerebral hematoma, are rare. Furthermore, only a few researchers have used 1H MRS for the detection of biochemical changes in the motor and motor association areas following the subordinate motor pathway damage (3, 23).
The biochemical information obtained during the present study shows that the M-1 NAA/Cr ratios are significantly decreased in the AH for intracerebral hematoma, which induces axonal injury to the internal capsule. The exact pathophysiologic mechanism of NAA loss in the normal-appearing motor cortex is unclear. However, reduced levels of NAA in normal-appearing white matter have already been shown to occur in those patients with multiple sclerosis and cerebral ischemia that has caused motor stroke (21, 24). We suggest that NAA loss in the M-1 in our present study may be caused by a retrograde degeneration according to the axonal injury at the level of internal capsule, and this is due to adjacent intracerebral hematoma. Pendlebury et al. (21) studied eighteen ischemic strokes that caused motor deficit with the use of 1H MRS, and they have shown that cortical and subcortical stroke induced NAA loss in the normal-appearing internal capsule. They explained this NAA loss in the internal capsule for the anterograde degeneration. The metabolic information obtained in our study shows that the NAA/Cr ratios in the M-1 are significantly decreased in the AH following an intracerebral hematoma. Although the examination time and the time associated with the disease are quite different, with respect to neural injury (i.e. the motor pathway), it is believed that there likely is a significant commonality between the two.
Reduced metabolism in the motor cortex has already been documented in a PET study dealing the ischemic stroke (25). In a PET study of 10 patients following recovery from striatocapsular infarction, the comparison of regional cerebral blood flow (rCBF) maps for patients at rest and normal subjects revealed the significantly lower regional cerebral blood flow in the basal ganglia, thalamus, sensorimotor, insular, and dorsolateral prefrontal cortices, in the brainstem, and in the ipsilateral cerebellum in patients, contralateral to the side of the recovered hand (25). These researchers suggest that the cause of decreased rCBF in these areas as a functional deactivation, although structural changes at a microscopic level due to transsynaptic or retrograde degeneration cannot be excluded. The reduction of the NAA/Cr ratios in the M-1 cortex in our study seems to be concordant with the already documented reduction of rCBF in the sensorimotor cortex by the PET study of recovered ischemic capsular infarction (25).
Puri et al. (3), evaluated the motor cortex by using in vivo 1H MRS in patients with incomplete spinal cord injury who showed a good motor function recovery. In that report, the mean level of the NAA/Cr ratio was significantly elevated in the motor cortex of patients, as compared with their ipsilateral occipital cortex or the cortical area of controls. They explained this increased NAA/Cr value as a neural adaptation, which accompanies recovery from spinal cord injury. At first glance, this increased NAA/Cr value in the motor cortex of the recovered incomplete spinal cord injury study seems to be contrary to our observations of decreased NAA/Cr values in the AH motor cortex with hemiparesis. However, there are several differences in the patients' characteristics, the methods used for MRS, location of voxels, etc. In a study by Puri et al. (3) the subjects were those who had already had shown good recovery of their motor function after neural injury, but we enrolled subjects who had not recovered from hemiparesis. In terms of the technical aspects of 1H MRS, Puri and his co-workers located the voxel in the centrum semiovale, and they used the technique of chemical shift imaging.
We found no significant correlation between the severity of weakness and the NAA/Cr values in the M-1, and we believe that the functional location of the voxel in our study was closer to the trunk and leg homunculus than the arm homunculus. Nevertheless, no correlation was found between the NAA/Cr values in the affected M-1 and the severity of leg weakness. However, we think it is difficult to draw a definite conclusion with our results because the number of patients enrolled in our study was so small and timing of the 1H MRS examination after the development of intracerebral hematoma was variable. At this time, it is not clear whether the NAA/Cr ratio of the AH would increase according to the recovery from hemiparesis in intracerebral hematoma. There is evidence that a significant recovery of NAA can occur after acute brain damage (19). For example, reversible decreases in NAA were found in two patients with mitochondrial encephalopathy and four patients with demyelinating lesions (24). Further study will be needed with a longer follow-up and larger numbers of subjects to investigate the correlation with the severity of weakness.
The SMA (M-II) occupies the medial surface of the superior frontal gyrus rostral to area 4, and this corresponds to the medial part of area 6 (area 6α) (26). The SMA is considered to be involved in the programming of learned skilled motor sequences and it may play a role in initiating voluntary movement (27). The recruitment of the SMA has been described as a normal accompaniment to either the anticipation or sequencing of a motor task (28). Clinical studies suggest that the SMA may contribute to the return of motor function after stroke (29, 30). Therefore, to investigate whether there is any biochemical alteration due to capsular injury in intracerebral hematoma, we included the bilateral SMA in addition to the M-1 in our study. In our present study, we found a significant reduction of the NAA/Cr ratios in both the AH and UH, as compared with the normal controls. It is difficult to explain the exact meaning of this reduction of NAA/Cr ratio in the bilateral SMAs. However, the SMA have striking bilateral influences upon both proximal and distal musculatures in primates, and cortical projections from the SMA are ipsilateral to areas 4-6, and 7, and to the contralateral SMA (26-28). Considering that the SMA has bilateral influence on motor function, we speculate that unilateral injury to the motor pathway might induce metabolic changes in the bilateral SMA by a possible retrograde degeneration.
In summary, we sought to determine whether 1H MRS is able to detect the metabolic changes of the M-1 and SMA that follow pyramidal tract injury after intracerebral hemorrhage. We found that the M-1 in the AH has lower NAA/Cr values than in the M-1 in the UH and in normal volunteers. In addition, the SMA also showed bilateral lower NAA/Cr values in both the AH and UH than in normal volunteers. Though we cannot precisely document the exact meaning of these lower NAA/Cr ratios, a presumed retrograde degeneration or functional deactivation may play a role in these NAA losses in M-1 and SMA due to motor tract injury. Further experiments should include longitudinal studies of patients with motor deficit after intracerebral hematomas, and whether there is a reversible component to this NAA loss.
Figures and Tables
Fig. 1
Voxel site for magnetic resonance spectroscopy (MRS) acquisition in the primary motor cortex (M-1) and supplementary motor area (SMA). (A) CT scan of a hemiparetic patient (Case 1). The intracerebral hematoma is in the left basal ganglia that is directly adjacent to the internal capsule. (B) M-1 voxel. Axial T2-weighted MR image showing a typical site of MRS acquisition of the M-1 cortex (rectangles). (C) SMA voxel. Axial T2-weighted MR image showing a typical site of MRS acquisition of the SMA cortex (rectangles).
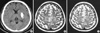
Fig. 2
Typical spectrum of proton magnetic resonance spectroscopy (1H MRS) in the chosen voxel. Graph showing typical MRS spectra obtained from the M-1 cortex in the affected and unaffected hemispheres (Case 1). All spectra were acquired using the STEAM technique; 20 msec TE, 2,000 msec TR, 128 averages, 2,500 Hz spectral width, and 2,048 data points.
(A) M-1 in affected hemisphere. (B) M-1 in unaffected hemisphere.
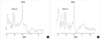
Table 1
Demographic data on patient's profile
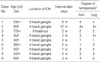
ICH, intracerebral hematoma; ictus, onset of bleeding; rt, right; lt, left; m, male; f, female; w, week; m, month. *Degree of hemiparesis, medical research council grading system (31).
Table 2
Comparison of metabolite ratios obtained by in vivo 1H MRS

*Values are expressed as means±SD. †shows significant differences when compared with control values (indepent sample t-test).
M-1, primary motor cortex; SMA, supplementary motor area; AH, affected hemisphere; UH, unaffected hemisphere; NAA, N-acetylaspartate; Cho, choline-containing compounds; Cr, creatine/phosphocreatine.
References
1. Rudkin TM, Arnold DL. Proton magnetic resonance spectroscopy for the diagnosis and management of cerebral disorders. Arch Neurol. 1999. 56:919–926.


2. Van der Knaap MS, van der Grond J, van Rijen PC, Faber JA, Valk J, Willemse K. Age-dependent changes in localized proton and phosphorus MR spectroscopy of the brain. Radiology. 1990. 176:509–515.


3. Puri BK, Smith HC, Cox IJ, Sargentoni J, Savic G, Maskill DW, Frankel HL, Ellaway PH, Davey NJ. The human motor cortex after incomplete spinal cord injury: an investigation using proton magnetic resonance spectroscopy. J Neurol Neurosurg Psychiatry. 1998. 65:748–754.


4. Berkelbach van der Sprenkel JW, Luyten PR, van Rijen PC, Tulleken CA, den Hollander JA. Cerebral lactate detected by regional proton magnetic resonance spectroscopy in a patient with cerebral infarction. Stroke. 1988. 19:1556–1560.


5. Bruhn H, Frahm J, Gyngell ML, Merboldt KD, Hanicke W, Sauter R. Cerebral metabolism in man after acute stroke: new observations using localized proton NMR spectroscopy. Magn Reson Med. 1989. 9:126–131.


6. Gideon P, Henriksen O, Sperling B, Christiansen P, Olsen TS, Jorgensen HS, Arlien-Soborg P. Early time course of N-acetyl aspartate, creatine and phosphocreatine, and compounds containing choline in the brain after acute stroke: a proton magnetic resonance spectroscopy study. Stroke. 1992. 23:1566–1572.
7. Federico F, Simone IL, Lucivero V, Giannini P, Laddomada G, Mezzapesa DM, Tortorella C. Prognostic value of proton magnetic resonance spectroscopy in ischemic stroke. Arch Neurol. 1998. 55:489–494.


8. Ford CC, Griffey RH, Matwiyoff NA, Rosenberg GA. Multivoxel 1H-MRS of stroke. Neurology. 1992. 42:1408–1412.
9. Gideon P, Sperling B, Arlien-Soborg P, Olsen TS, Henriksen O. Long-term follow-up of cerebral infarction patients with proton magnetic resonance spectroscopy. Stroke. 1994. 25:967–973.


10. Graham GD, Kalvach P, Blamire AM, Brass LM, Fayad PB, Prichard JW. Clinical correlates of proton magnetic resonance spectroscopy findings after acute cerebral infarction. Stroke. 1995. 26:225–229.


11. Frahm J, Bruhn H, Gyngell ML, Merboldt KD, Hanicke W, Sauter R. Localized high-resolution proton NMR spectroscopy using stimulated echoes: Initial applications to human brain in vivo. Magn Reson Med. 1989. 9:79–93.
12. Frahm J, Merbold KD, Hanicke W. Localized proton spectroscopy using stimulated echoes. J Magn Reson. 1987. 72:502–508.


13. Kreis R, Farrow N, Ross BD. Localized 1H NMR spectroscopy in patients with chronic hepatic encephalopathy: Analysis of changes in cerebral glutamine, choline, and inositols. NMR Biomed. 1991. 4:109–116.
14. Petroff OA, Spencer DD, Alger JR, Prichard JW. High-field proton magnetic resonance spectroscopy of human cerebrum obtained during surgery for epilepsy. Neurology. 1989. 39:1197–1202.


15. Gresham GE, Duncan PW, Stason WB. Post-stroke Rehabilitation. 1995. Rocksville, MD: U.S. Department of Health and Human Services. Public Health Services, Agency for Health Care Policy and Research.
16. Tallan HH, Moore S, Stein WH. N-acetyl-L-aspartic acid in brain. J Biol Chem. 1956. 219:257–264.


17. Urenjak J, Williams SR, Gadian DG, Noble M. Specific expression of N-acetyl aspartate in neurons, oligodendrocyte-type-2 astrocyte progenitors and immature oligodendrocytes in vitro. J Neurochem. 1992. 59:55–61.
18. Ross B, Michaelis T. Clinical applications of magnetic resonance spectroscopy. Magn Reson Q. 1994. 10:191–247.
19. De Stefano N, Matthews PM, Arnold DL. Reversible decrease in N-acetylaspartate after acutre brain injury. Magn Reson Med. 1995. 34:721–727.
20. Bates TE, Strangward M, Keelan J, Davey GP, Munro PM, Clark JB. Inhibition of N-acetylaspartate production: implications for 1H MRS studies in vivo. Neuroreport. 1996. 7:1397–1400.
21. Pendlebury ST, Blaire AM, Lee MA, Styles P, Matthews PM. Axonal injury in the internal capsule correlates with motor impairment after stroke. Stroke. 1999. 30:956–962.


22. Fu L, Matthews PM, De Stefano N, Worsley KJ, Narayanan S, Francis GS, Antel JP, Wolfson C, Arnold DL. Imaging axonal damage of normal-appearing white matter in multiple sclerosis. Brain. 1998. 121:103–113.


23. Pioro EP, Antel JP, Cashman NR, Arnold DL. Detection of cortical neuron loss in motor neuron disease by proton mangnetic resonance spectroscopic imaging in vivo. Neurology. 1994. 44:1933–1938.
24. Husted CA, Goodin DS, Hugg JW, Maudsley AA, Tsuruda JS, de Bie SH, Fein G, Matson GB, Weiner MW. Biochemical alterations in multiple sclerosis lesions and normal appearing white matter detected by in vivo 31P and 1H spectroscopic imaging. Ann Neurol. 1994. 36:157–165.
25. Weiller C, Chollet F, Friston KJ, Wise RJ, Frackowiak RS. Functional reorganization of the brain recovery from striatocapsular infarction in man. Ann Neurol. 1992. 31:463–472.
26. Jones EG. Jones EG, Peters A, editors. Connectivity of primate sensory-motor cortex. Cerebral cortex. 1986. Vol. 5. New York: Plenum Press;113–183.
27. Wiesendanger M. Recent developments in studies of the supplementary motor area of primates. Rev Physiol Biochem Pharmacol. 1986. 103:1–59.


28. Marsden CD, Deecke L, Freund H-J, Hallet M, Passingham RE, Shibasaki H, Tanji J, Wiesendanger M. The functions of the supplementary motor area. Summary of a workshop. Adv Neurol. 1996. 70:477–487.
29. Fries W, Danek A, Schneidtmann K, Hamburger C. Motor recovery following capsular stroke: role of descending pathways from multiple motor areas. Brain. 1993. 116:369–382.
30. Cramer SC, Nelles G, Benson RR, Kaplan JD, Parker RA, Kwong KK, Kennedy DN, Finklestein SP, Rosen BR. A functional MRI study of subjects recovered from hemiparetic stroke. Stroke. 1997. 28:2518–2527.


31. Medical Research Council. Memorandum no. 45. Aid to examination of the peripheral nervous system. 1976. London: Her Majesty's Stationery Office.