Abstract
Transforming growth factor (TGF)-β1 is an important fibrogenic factor that is involved in the pathogenesis of diabetic nephropathy. We evaluated the effect of circular antisense TGF-β1 oligodeoxynucleotides (ODNs) on the TGF-β1 expression in the rat mesangial cell culture and in streptozotocin (STZ)-induced diabetic rats. Circular antisense TGF-β1 ODNs were found to be stable in rat serum, significantly decreasing TGF-β1 mRNA expression compared with linear antisense ODNs in the rat mesangial cell culture. Circular antisense TGF-β1 ODNs were introduced into the tail vein of normal rats using hemagglutinating virus of Japan (HVJ)-liposome-mediated gene transfer method and were confirmed to be delivered effectively into the kidney, liver, lungs, and spleen. To inhibit the overexpression of TGF-β1 in diabetic kidneys, we introduced circular antisense TGF-β1 ODNs into the STZ-induced diabetic rats. On day 13 after circular antisense TGF-β1 ODNs injection, TGF-β1 mRNA and protein expression markedly decreased and urinary TGF-β1 excretion rate also dropped in the circular antisense TGF-β1 ODNs-treated diabetic rats. These results suggest that circular antisense TGF-β1 ODNs may be a useful tool for developing new therapeutic application for progressive diabetic nephropathy.
Diabetic nephropathy is the most common cause of end stage renal disease in developed countries and a major cause of morbidity and mortality in patients with diabetes. It is characterized by structural abnormalities including hypertrophy of both glomerular and tubular elements, increase in the thickness of glomerular basement membranes, and progressive accumulation of extracellular matrix components. It also results in functional alterations including the early increase in the glomerular filtration rate with intraglomerular hypertension, subsequent proteinuria, systemic hypertension, and eventual loss of renal function (1-4). The development of irreversible renal change in diabetes mellitus such as glomerulosclerosis and tubulointerstitial fibrosis results ultimately in renal failure.
It has been established that transforming growth factor (TGF)-β1 is a critical cytokine involved in diabetes-induced extracellular matrix accumulation in both cell culture and animal models of diabetic kidney disease. Investigations of glomerular mesangial cells and proximal tubular cells have demonstrated that high glucose media induce the enhanced expression of TGF-β1 mRNA and protein and the biosynthesis of collagen and other extracellular matrix constituents (5-9). Several in vivo studies have also reported that TGF-β1 expression is elevated in diabetic kidneys (10-14). TGF-β is a multifunctional cytokine that plays an important role in healing wounds and repairing tissues. In mammals the cytokine has three isoforms i.e., TGF-β1, 2, and 3, whose biological properties are nearly identical. Among the three isoforms, TGF-β1 is known to contribute mostly to the pathogenesis of tissue fibrosis of organs such as kidney, liver, lungs, and heart and scarring of superficial tissues. TGF-β1 plays a central role in tissue repair by stimulating the balanced expression of extracellular matrix such as collagen, fibronectin, and matrix proteoglycans. However, persistent injury with sustained autoinduction of TGF-β1 overrides normal termination signals and leads to the continuous production of TGF-β1 and extracellular matrix; thus resulting in tissue fibrosis (15, 16). Therefore, anti-TGF-β1 or anti-fibrotic therapies have been attempted by administering various reagents: anti-TGF-β1 antibody, interferon-α, anti-oxidants such as α-tocopherol, and decorin (17-20).
In diabetic kidney models, anti-TGF-β1 antibodies significantly suppressed renal hypertrophy and expression of genes encoding extracellular matrix components (19). Therapy with antisense TGF-β1 oligodeoxynucleotides (ODNs) decreased TGF-β1 production and reduced hyperglycemia-induced proximal tubular cell hypertrophy in vitro and partially prevented the increase in kidney weight and extracellular matrix expression (21). Anti-TGF-β1 therapies on diabetic models to date suggested that blockade of the biological actions of TGF-β1 may be useful in preventing matrix accumulation at the early stage of diabetic nephropathy.
In recent years, gene therapy has been tried actively with antisense ODNs to block the aberrant gene expression implicated in many diseases. Antisense ODNs can work through several potential mechanisms involving hybridization with mRNA and function of RNase H that has the ability to degrade the target message (22). However, biological instability is the first problem to consider when delivering ODNs to cells. Unmodified phosphodiester backbone ODNs are rapidly degraded in the biological fluid by a combination of both endo- and exonucleases. To overcome this problem, a variety of chemically modified ODNs have been developed: phosphorothioates, methylphosphonates, and phosphoramidate analogues (22-25). Nonetheless, most of the modified ODNs exhibited problems as well. Phosphorothioate ODNs have non-sequence related toxicity, while methylphosphonate ODNs show resistance to RNase H (22, 26). Compared with natural DNA/RNA complexes, phosphoramidate oligonucleotides are weak activators of RNase H (22). Given these problems, circular antisense ODNs only structurally modified for stability against nucleases have been developed recently (27). These were in contrast with those of other chemically modified ODNs, designed only through structural modification.
The aim of the present study was to investigate the effect of circular antisense TGF-β1 ODNs on the TGF-β1 expression in the rat mesangial cell culture and in streptozotocin (STZ)-induced diabetic rats.
Circular antisense TGF-β1 ODNs were synthesized as described previously (27, 28). The selection of antisense ODNs for TGF-β1 was performed using the DNAsis program (Hitach Software, San Bruno, CA, U.S.A.). The sequences of ODN for rat TGF-β1 mRNA (755 to 800 of the rat TGF-β1 mRNA sequence, GenBank, accession X52498) are as follows:
Antisense, 5'-TGTGTGTGATGTCTTTGGTTTTGTCATAGATTTCGTTGTTGCGGTC-3';
Sense, 5'-GACCGCAACAACGCAATCTATGACAAAACCAAAGACATCACACACA-3'.
To form a closed structure, both antisense ODNs and sense ODNs were modified. The 5' end of ODNs has 8 bases of sequence of 5'-GATCACGT-3', while the 3' end of ODNs has 4 bases of sequence of 5'-ACGT-3'. The modified 58-nucleotides (nt) ODNs were custom-synthesized by Bionics (Seoul, Korea). The linear 58-nt ODNs were joined by the complementary 4 base sequences (5'-GATC-3') at the 5' ends. Briefly, 60 µg of linear antisense TGF-β1 ODNs were incubated in a final volume of 100 µL containing 1× ligase buffer and 3,500 U T4 DNA ligase (Takara, Otsu, Japan) at 16℃ for 24 hr. The formation of circular ODNs (116-nt) was confirmed by electrophoresis on a 6% denaturing polyacrylamide gel containing 7 M urea.
Circular antisense TGF-β1 ODNs and linear antisense ODNs were incubated at 37℃ for 24 hr with rat serum which was not inactivated by heat to preserve nuclease activity. The serum was added to ODNs in a 50% volume of reaction mixture. ODNs were then extracted with phenol/chloroform (1:1, v/v) and examined by electrophoresis on a 6% denaturing polyacrylamide gel containing 7 M urea.
Rat mesangial cells (RMCs) were obtained from a culture of glomeruli isolated from male Sprague-Dawley (SD) rats weighing 100 to 150 g using differential sieving methods as previously described (29). Isolated glomeruli were cultured in RPMI 1640 (Gibco BRL, Gaithersburg, MD, U.S.A.) complete medium containing 100 U/mL penicillin, 100 µg/mL streptomycin, 0.25 µg/mL amphotericin B, 10 mg/L insulin, 6.7 µg/L sodium selenite, 5.5 mg/L transferrin, and 20% heat-inactivated fetal calf serum (FCS; HyClone, Logan, UT, U.S.A.) at 37℃ in a humidified 5% CO2 atmosphere. The identity of mesangial cells was confirmed by phase contrast microscopy according to the morphologic criteria (30). RMCs between the 10th and 15th passage were used for cell studies.
RMCs were seeded at 2×104 cells/well in 8-well chamber slides (Nunc, Inc., Naperville, IL, U.S.A.) and cultured in RPMI 1640 complete medium for 24 hr. RMCs were serum-starved for 48 hr in RPMI 1640 containing 0.5% FCS and transfected with fluorescein-labeled-circular antisense TGF-β1 ODNs. Circular antisense TGF-β1 ODNs were labeled using Label IT® Fluorescein Nucleic Acid Labeling Kit (Panvera Corp., Madison, WI, U.S.A.). Commercially available cationic liposomes (LipofectAMINE PLUS™ Reagent; Gibco BRL, Gaithersburg, MD, U.S.A.) were used to facilitate the transfection of ODNs into RMCs. Briefly, 0.5 µg of ODNs in DMEM (Gibco BRL, Gaithersburg, MD, U.S.A.) were mixed with the Plus reagent and incubated at room temperature for 15 min. The ODNs-Plus reagent were mixed with 2 µg of LipofectAMINE reagent and subsequently incubated at room temperature for 15 min. The cells were washed with serum-free DMEM and then 0.03 mL/well of serum-free DMEM was added to the cells. The ODNs-liposome complexes were added drop by drop to each well and incubated at 37℃ for 5 hr. Cells were then replaced with RPMI 1640 containing 17% FCS and incubated further at 37℃ for 24 hr. Finally, the RMCs were washed three times with phosphate-buffered saline (PBS) and fixed in methanol at 4℃ for 10 min. The cells were mounted and observed through a fluorescence microscope. The effect of antisense ODNs on TGF-β1 mRNA expression in RMCs was investigated. Cells were cultured at 2×105 cells/well in 6-well cell culture plates (Nunc, Inc., Naperville, IL, U.S.A.) and circular antisense (0.5 µg or 1 µg), linear antisense (0.5 µg or 1 µg), and circular sense (1 µg) TGF-β1 ODNs were introduced selectively into the cells as described above.
The hemagglutinating virus of Japan (HVJ)-liposomes were prepared as described previously (31) with minor modifications. HVJ was grown in chorioallantoic fluid of 10-day-old embryonated chicken eggs at 35.5℃. HVJ was collected by centrifugation at 3,000 rpm for 10 min and suspended with balanced salt solution (BSS; 140 mM NaCl, 5.4 mM KCl, 10 mM Tris-HCl, pH 7.5). HVJ was purified by another centrifugation at 12,000 rpm for 1 hr, resuspended with BSS, and stored at 4℃ until it was used. Egg yolk phosphatidyl choline (ePC; Sigma, U.S.A.), dioleoyl phosphatidyl ethanolamine (DOPE; Avanti Polar Lipid, Birmingham, AL, U.S.A.), egg yolk sphingomyelin (eSph; Sigma, U.S.A.), bovine brain phosphatidyl serine (bPS; Sigma, U.S.A.), and cholesterol (Chol; Sigma, U.S.A.) were each dissolved in chloroform, mixed in a weight ratio of 1.6:3:1.5:1.3:1.5, and then dried with a rotary evaporator. The dried lipid mixture was hydrated in 200 µL of BSS containing 10 µg of the ODNs, mixed with vigorous agitation, and filtered to form liposomes. The liposomes were mixed with HVJ that had been inactivated by ultraviolet irradiation. The mixture was incubated at 4℃ for 10 min and then at 37℃ for 60 min with gentle agitation. Free HVJ was removed from the HVJ-liposome mixture by sucrose density gradient centrifugation. The HVJ-liposome suspensions were maintained at 4℃ until it was used.
To estimate the delivery efficiency of the circular antisense TGF-β1 ODNs by intravenous administration, fluorescein-labeled-circular antisense TGF-β1 ODNs were injected into the tail vein of normal 6-week-old male SD rats by the HVJ-liposome mediated gene transfer method. Kidney, liver, lungs, and spleen were removed 24 hr after injection. Four-µm thick cryostat sections of unfixed snap-frozen specimens were observed through a confocal laser scanning microscope (TCS/SPII, Leica, Wetzlar, Germany).
Diabetes was induced in 21 male SD rats (180-210 g) by a single intraperitoneal injection of 70 mg/kg STZ (Sigma, U.S.A.) dissolved in 10 mM sodium citrate buffer (pH 4). Blood glucose was measured using reagent strips (MediSense, Bedford, MA, U.S.A.) 48 hr after STZ administration. Animals with blood glucose >15 mM were included in this study. All of the animals had unlimited access to standard rat food and water. STZ-induced diabetic rats were separated into two groups. One group (ODNs-treated diabetic rats) was treated with circular antisense TGF-β1 ODNs and the other group (untreated diabetic rats) was not. Once hyperglycemia was detected, HVJ-liposome mediated circular antisense TGF-β1 ODNs were injected into the tail vein of the diabetic rat the following day. Each group was sacrificed on day 1, 5, and 13 after injection of ODNs. Normal rats were sacrificed on day 13. Rats were anesthetized with ethyl ether and the kidneys were removed, immediately frozen in liquid nitrogen, and stored at -70℃ for subsequent RNA extraction. Portions of tissues were fixed in neutral buffered formalin for immunohistochemical staining. At the end of each experimental periods, individually collected urine was centrifuged at 3,000 rpm at 4℃ for 10 min. The supernatant was collected and stored at -70℃ until it was used.
Total RNA was extracted from cultured RMCs and frozen kidneys with RNAzol B (TEL-TEST, Friendswood, TX, U.S.A.) according to the manufacturer's instructions. The purity and quantity of the RNA preparation were determined by measuring the optical densities at 260 and 280 nm. Total RNA was reverse transcribed with oligo-d(T)15 primers and M-MLV reverse transcriptase (Promega, Madison, WI, U.S.A.). Aliquots of cDNA were amplified by PCR using primer sets specific to rat TGF-β1 and a constitutively expressed housekeeping gene, glyceraldehyde-3-phosphate-dehydrogenase (GAPDH) as the control. The primers used for PCR are as follows:
Rat TGF-β1 upstream CCTGCTGCTTTCTCCCTCAACC, downstream CTGGCACTGCTTCCCGAATGTC;
Rat GAPDH upstream GTGGACATTGTTGCCATCAACG, downstream GAGGGAGTTGTCATATTTCTCG.
The reaction was run for 25 cycles with a cycling parameter of 30 sec at 95℃, 60 sec at 60℃, and 120 sec at 72℃. PCR products were visualized on a 2% agarose gel. The amounts of TGF-β1 mRNA were measured by densitometer and expressed relative to the densities of GAPDH.
Paraffin-embedded sections obtained from the kidneys were deparaffinized with xylene and graded ethanol solutions and incubated in 3% H2O2 in methanol at room temperature for 20 min to block endogenous peroxidase activity. They were then washed with PBS for 10 min, incubated at 37℃ for 30 min in 0.1% trypsin, and washed with PBS for 5 min three times. Incubation with the first antibody (Rabbit anti-porcine TGF-β1 polyclonal antibody; Cell Science, Norwood, MA, U.S.A.) was performed at 37℃ for 1 hr. After three serial washes with PBS, the sections were processed by an indirect immunoperoxidase technique using a commercial kit (LSAB kit; DAKO, Carpinteria, CA, U.S.A.), counterstained with Mayer's hematoxylin, and examined under light microscope.
Total TGF-β1 in rat urine was determined using a sandwich ELISA kit (TGF-β1 Emax ImmunoAssay System; Promega, Madison, WI, U.S.A.), following the instructions provided by the manufacturer (32). Acid activation of urine samples was required to convert latent TGF-β1 into active form and record detectable levels of total TGF-β1. Samples were activated with 1N HCl at room temperature for 30 min, followed by neutralization with 1N NaOH. Samples were plated on microtiter plates coated with anti-TGF-β1 monoclonal antibody and incubated at room temperature for 3 hr with shaking. After vigorous washing, wells were incubated overnight with polyclonal anti-TGF-β1 at 4℃ and washed. They were then incubated with antibody conjugated with horseradish peroxidase at room temperature for 3 hr with shaking. Following additional washes, color was developed by adding peroxidase substrate in 3,3',S,S'-tetramethyl benzidine solution. A standard curve was constructed using serial dilutions of human TGF-β1. TGF-β1 levels in the samples were determined from the standard curve.
Circular antisense TGF-β1 ODNs (116-nt) synthesized by T4 DNA ligase from linear ODNs (58-nt) and analyzed by denaturing polyacrylamide gel electrophoresis had slower mobility compared with linear ODNs (Fig. 1A).
This test was performed to prove the stability of ODNs against nucleases in serum. Linear antisense TGF-β1 ODNs were completely digested after incubation for 24 hr in rat serum. However, circular antisense TGF-β1 ODNs remained considerably intact after incubation for 24 hr in rat serum; thus exhibiting markedly improved stability compared with linear antisense ODNs (Fig. 1B).
To examine the transfection efficiency and localization of circular antisense TGF-β1 ODNs, fluorescein-labeled-circular antisense TGF-β1 ODNs were transfected to RMCs. The cells were then evaluated by fluorescence microscopy. Most of the RMCs exhibited strong fluorescence in the cytoplasm and nucleus (Fig. 2).
To examine the effect of antisense ODNs, circular antisense ODNs (0.5 µg or 1 µg), linear antisense ODNs (0.5 µg or 1 µg), and circular sense TGF-β1 ODNs (1 µg) were introduced selectively into the RMCs. Total RNA was isolated from the transfected cells and RT-PCR was performed. Compared with the transfection of circular sense TGF-β1 ODNs (1 µg) and liposome alone, the transfection of circular antisense TGF-β1 ODNs reduced TGF-β1 mRNA levels. Furthermore, the effect of circular antisense ODNs on the ablation of the TGF-β1 mRNA content was stronger than that of the linear antisense ODNs in the same amount of ODNs. These results indicated that circular antisense TGF-β1 ODNs were more effective than linear antisense ODNs in reducing TGF-β1 mRNA expression (Fig. 3).
To evaluate the efficiency of circular antisense TGF-β1 ODNs via intravenous administration, fluorescein-labeled-circular antisense TGF-β1 ODNs were introduced into the tail vein of normal SD rats using the HVJ-liposome transfer method. The kidney, liver, lungs, and spleen were removed 24 hr after the tail vein injection of ODNs and observed through a confocal laser scanning microscope. Most of the tubular epithelial cells exhibited strong fluorescence in the cytoplasms and nuclei (Fig. 4A). In addition, results confirmed that ODNs were delivered mostly into liver, lungs, and spleen (Fig. 4B-D).
Table 1 shows the parameters measured during the induction of diabetes. Both circular antisense TGF-β1 ODNs-treated diabetic rats and untreated diabetic rats had slightly higher blood glucose level on day 13 after ODNs injection compared to days 1 and 5. Treatment with circular antisense TGF-β1 ODNs had little effect on the blood glucose level of diabetic rats, however.
The effect of circular antisense ODNs on TGF-β1 mRNA and protein expression in diabetic kidneys was determined by RT-PCR and immunohistochemical study. Circular antisense TGF-β1 ODNs-treated and untreated diabetic rats were studied on days 1, 5, and 13 after the introduction of ODNs. On day 13 after ODNs injection, TGF-β1 mRNA levels were decreased significantly in circular antisense TGF-β1 ODNs-treated diabetic rats compared with untreated diabetic rats (0.82±0.05 vs. 1.32±0.01; p<0.05) (Fig. 5). Furthermore, circular antisense TGF-β1 ODNs treatment markedly inhibited TGF-β1 protein expression in diabetic kidneys (Fig. 6). On days 1 and 5 after ODNs injection, TGF-β1 was strongly expressed in the glomerular endothelial cells and tubular epithelial cells of the untreated diabetic kidneys (Fig. 6A, C), while circular antisense TGF-β1 ODNs-treated diabetic kidneys (Fig. 6B, D) showed mild TGF-β1 protein expression in the tubular epithelial cells. On day 13 after ODNs injection, TGF-β1 was markedly expressed in the peritubular capillaries, glomerular endothelial cells, and tubular epithelial cells of the untreated diabetic kidneys compared with the circular antisense TGF-β1 ODNs-treated diabetic kidneys (Fig. 6E-G).
Urinary excretion rate of total TGF-β1 (latent plus active fractions) markedly increased in both untreated and circular antisense TGF-β1 ODNs-treated diabetic rats. On days 1 and 5 after ODNs injection, the urinary TGF-β1 excretion rates of circular antisense TGF-β1 ODNs-treated diabetic rats were not significantly different from that of the untreated diabetic rats. In contrast, on day 13 after ODNs injection, the urinary TGF-β1 excretion rate of circular antisense TGF-β1 ODNs-treated diabetic rats showed approximately 25% reduction compared with that of the untreated diabetic rats (Fig. 7).
Much attention has been focused on exploring mechanisms related to the development of diabetic nephropathy. Among the mechanisms, TGF-β1 has been implicated as an etiologic cause in its progression. TGF-β1 is a key mediator of the hyperglycemia-induced panoramic effects on cell growth and extracellular matrix accumulation (7, 9-11, 13, 14). In addition, this cytokine has powerful fibrogenic potential because of its capability for simultaneous actions such as stimulation of matrix synthesis, inhibition of matrix degradation, and modulation of matrix receptor expression to facilitate cell matrix interactions (16). Thus, TGF-β1 has been considered as a therapeutic target in fibrotic disease such as diabetic nephropathy and other chronic kidney diseases, and anti-TGF-β1 therapies have also been performed (17-20).
More recently, gene therapy using antisense ODNs as one of the anti-TGF-β1 therapies has been investigated and applied to renal disease (21, 31, 33). In the period of early gene therapy, a major problem was the rapid degradation of phosphodiester antisense ODNs by nuclease both in vitro and in vivo. To improve the biological instability of antisense ODNs, chemical modifications of the phosphate backbone or bases were attempted and ODNs such as phosphorothioate, methylphosphate, and phosphoramidate analogues have been developed (22). In particular, phosphorothioate (PS)-ODNs have been studied mainly in animal models of kidney disease where one of the non-bridging oxygens in the phosphodiester backbone is replaced with a sulfur atom. However, PS-ODNs were found to have non-sequence-specific effects because of their polyanionic nature (25, 26).
Circular antisense TGF-β1 ODNs used in the present work were synthesized from linear antisense ODNs, retaining the integrity of the phosphodiester backbone of the ODNs for its stability to nuclease attack. Theoretically, these ODNs could resist exonuclease attack with excellent binding affinity, sequence specificity, and ability to activate RNase H since they possess no 5' or 3'end. In this study, it was shown that circular antisense ODNs in rat serum were less digested than linear ODNs. The ablation of the TGF-β1 mRNA was more marked in the circular antisense ODNs than in the linear antisense ODNs treated RMCs. These results indicate that circular antisense TGF-β1 ODNs are superior to linear antisense ODNs. Furthermore, circular antisense TGF-β1 ODNs markedly suppressed the levels of TGF-β1 mRNA and protein in diabetic kidneys. Its specific activity in blocking TGF-β1 expression was demonstrated by the observed absence of difference in the level of GAPDH mRNA. An enhanced stability of the circular antisense TGF-β1 ODNs could enable less frequent dosing because of its longer duration of action, thereby reducing potential unwanted side-effects from fewer degradation metabolites.
Various routes for the efficient transfer of ODNs into the kidney have been tried in experimental models. Akagi et al. (31) reported transfection of ODNs via renal artery in the anti-Thy glomerulonephritis model. Isaka et al. (33) introduced retrograde transfection of ODNs via the ureter in the unilateral ureter obstruction model. Han et al. (21) reported systemic infusion of ODNs through implanted osmotic pump in diabetic mice. All these works should be performed with minor-surgery for administration and would be quite troublesome in case of repetitive administration. Morishita et al. (34) attempted systemic administration via the tail vein for insulin vector transfer, resulting in the expression of insulin vector in the liver and spleen. Others reported that circulating ODNs could reach and accumulate in the kidney (35, 36). With those reports taken into consideration, the present study used intravenous administration to transfer ODNs to the kidney. After the intravenous administration, circular antisense TGF-β1 ODNs appeared in the liver, lungs, spleen, and kidney. In the kidneys, they appeared mainly in the tubular epithelial cells. In addition, they inhibited effectively the expression of TGF-β1 mRNA and protein in diabetic kidneys. These results are consistent with the notion that systemic administration of antisense TGF-β1 ODNs is effective in reducing TGF-β1 expression (21). The study also employed HVJ-liposome method for the effective delivery of ODNs into kidney cells because of the several advantages of this delivery system, such as rapid translocation to the nucleus and stability of the transfected ODNs in the nucleus (37).
In the long-term diabetic state, TGF-β1 may perpetuate the disease process by inducing persistent hyperglycemia. In this regard, it seems necessary to perform further studies on the long-term blockade of progression of diabetic nephropathy and renal failure through treatment with circular antisense TGF-β1 ODNs.
In conclusion, the effect of circular antisense TGF-β1 ODNs designed for stability against nucleases was superior to that of the linear antisense ODNs on TGF-β1 mRNA expression in RMCs. Circular antisense TGF-β1 ODNs suppressed efficiently the expression of TGF-β1 in the mRNA and protein levels by intravenous administration combined with HVJ-liposome in STZ-induced diabetic rats. These findings suggest that circular antisense TGF-β1 ODNs may be a useful tool for developing new therapeutic application for progressive diabetic nephropathy.
Figures and Tables
Fig. 1
Photograph of stained 6% denaturing polyacrylamide gel showing relative mobilities of linear and circular antisense TGF-β1 oligodeoxynucleotides (ODNs). (A) Synthesis of circular antisense TGF-β1 ODNs from linear ODNs: lane 1, 58-nt linear antisense TGF-β1 ODNs; lane 2, 116-nt circular antisense TGF-β1 ODNs. (B) Stability of circular and linear antisense TGF-β1 ODNs after the treatment with rat serum: lane 1, untreated circular antisense TGF-β1 ODNs; lane 2, circular antisense TGF-β1 ODNs treated with 50% (v/v) rat serum for 24 hr; lane 3, untreated linear antisense TGF-β1 ODNs; lane 4, linear antisense TGF-β1 ODNs treated with 50% (v/v) rat serum for 24 hr.
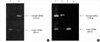
Fig. 2
Fluorescence microscopy of rat mesangial cells transfected with circular antisense TGF-β1 oligodeoxynucleotides (ODNs) in cationic liposomes. Fluorescein-labeled-circular antisense TGF-β1 ODNs were observed in both the nuclei and the cytoplasm (×400).

Fig. 3
Effect of circular antisense oligodeoxynucleotides (ODNs) on TGF-β1 mRNA expression in rat mesangial cells (RMCs). RMCs were serum-starved for 48 hr and subsequently transfected with circular antisense ODNs (1 µg)+cationic liposome (4 µg) (lane 1), circular antisense ODNs (0.5 µg)+cationic liposome (4 µg) (lane 2), linear antisense ODNs (1 µg)+cationic liposome (4 µg) (lane 3), linear antisense ODNs (0.5 µg)+cationic liposome (4 µg) (lane 4), circular sense ODNs (1 µg)+cationic liposome (4 µg) (lane 5), and cationic liposome (4 µg) alone (lane 6), and then exposed to RPMI 1640 containing 17% FCS. Total RNA was isolated from the RMCs and subjected to RT-PCR (TGF-β1: 598 bp, GAPDH: 351 bp).
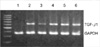
Fig. 4
Confocal microscopy of rat organs transfected with fluorescein-labeled-circular antisense TGF-β1 ODNs in HVJ-liposome by intravenous systemic administration method (A: kidney, B: liver, C: lung, D: spleen, ×200).
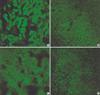
Fig. 5
RT-PCR analysis of the effect of circular antisense oligodeoxynucleotides (ODNs) on TGF-β1 mRNA expression in diabetic kidneys on days 1, 5, and 13 after injection of ODNs into diabetic rats. Lane 1, normal kidney; lane 2, on day 1, untreated diabetic kidney; lane 3, on day 1, ODNs-treated diabetic kidney; lane 4, on day 5, untreated diabetic kidney; lane 5, on day 5, ODNs-treated diabetic kidney; lane 6, on day 13, untreated diabetic kidney; lane 7, on day 13, ODNs-treated diabetic kidney (TGF-β1: 598 bp, GAPDH: 351 bp).

Fig. 6
Immunohistochemical staining of TGF-β1. On day 1 after injection of circular antisense TGF-β1 oligodeoxynucleotides (ODNs) into diabetic rats, ODNs-treated diabetic kidney (B), on day 5, ODNs-treated diabetic kidney (D), on day 13, ODNs-treated diabetic kidney (G), and their respective controls (A, C, E, F: untreated diabetic kidney) (×200).

Fig. 7
Effect of circular antisense oligodeoxynucleotides (ODNs) on urinary TGF-β1 excretion rate. Collected urine samples on days 1, 5, and 13 after injection of ODNs into diabetic rats were assayed by a TGF-β1 ELISA (normal: open bars, diabetes: hatched bars, ODNs-treated diabetes: solid bars). Data are expressed as mean±SE. *: p<0.05 (normal vs. diabetes).
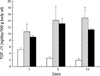
ACKNOWLEDGMENTS
A part of the technique used in this experiment was supported by Welgene biopharmaceuticals Inc.. This work was supported by Korean Research Foundation Grant (KRF-2002-005-E00005).
References
1. Ziyadeh FN, Goldfarb S. The renal tubulointerstitium in diabetes mellitus. Kidney Int. 1991. 39:464–475.


2. Ziyadeh FN. Renal tubular basement membrane and collagen type IV in diabetes mellitus. Kidney Int. 1993. 43:114–120.


4. Bertoluci MC, Schmid H, Lachat JJ, Coimbra TM. Transforming growth factor-beta in the development of rat diabetic nephropathy. A 10-month study with insulin-treated rats. Nephron. 1997. 74:189–196.
5. Rocco MV, Chen Y, Goldfarb S, Ziyadeh FN. Elevated glucose stimulates TGF-β gene expression and bioactivity in proximal tubule. Kidney Int. 1992. 41:107–114.


6. Wolf G, Sharma K, Chen Y, Ericksen M, Ziyadeh FN. High glucose-induced proliferation in mesangial cells is reversed by autocrine TGF-β. Kidney Int. 1992. 42:647–656.


7. Ziyadeh FN, Sharma K, Ericksen M, Wolf G. Stimulation of collagen gene expression and protein synthesis in murine mesangial cells by high glucose is mediated by autocrine activation of transforming growth factor-β. J Clin Invest. 1994. 93:536–542.
8. Hoffman BB, Sharma K, Zhu Y, Ziyadeh FN. Transcriptional activation of transforming growth factor-β1 in mesangial cell culture by high glucose concentration. Kidney Int. 1998. 54:1107–1116.


9. Oh JH, Ha HJ, Yu MR, Lee HB. Sequential effects of high glucose on mesangial cell transforming growth factor-β1 and fibronectin synthesis. Kidney Int. 1998. 54:1872–1878.


10. Ihm CG, Lee GS, Nast CC, Artishevsky A, Guillermo R, Levin PS, Glassock RJ, Adler SG. Early increased renal procollagen α1 (IV) mRNA levels in streptozotocin induced diabetes. Kidney Int. 1992. 41:768–777.
11. Bollineni JS, Reddi AS. Transforming growth factor-β1 enhances glomerular collagen synthesis in diabetic rats. Diabetes. 1993. 42:1673–1677.


12. Shankland SJ, Scholey JW, Ly H, Thai K. Expression of transforming growth factor-β1 during diabetic renal hypertrophy. Kidney Int. 1994. 46:430–442.


13. Park IS, Kiyomoto H, Abboud SL, Abboud HE. Expression of transforming growth factor β and type IV collagen in early streptozotoc-ininduced diabetes. Diabetes. 1997. 46:473–480.
14. Hill C, Flyvbjerg A, Gronbaek H, Petrik J, Hill DJ, Thomas CR, Sheppard MC, Logan A. The renal expression of transforming growth factor-β isoforms and their receptors in acute and chronic experimental diabetes in rats. Endocrinology. 2000. 141:1196–1208.


15. Roberts AB, McCune BK, Sporn MB. TGF-β: regulation of extracellular matrix. Kidney Int. 1992. 41:557–559.


16. Border WA, Noble NA. Transforming growth factorβ in tissue fibrosis. N Engl J Med. 1994. 331:1286–1292.
18. Border WA, Noble NA, Ketteler M. TGF-β: a cytokine mediator of glomerulosclerosis and a target for therapeutic intervention. Kidney Int Suppl. 1995. 49:S59–S61.
19. Sharma K, Jin Y, Guo J, Ziyadeh FN. Neutralization of TGF-β by anti-TGF-β antibody attenuates kidney hypertrophy and the enhanced extracellular matrix gene expression in STZ-induced diabetic mice. Diabetes. 1996. 45:522–530.


20. Border WA, Noble NA. TGF-β in kidney fibrosis: a target for gene therapy. Kidney Int. 1997. 51:1388–1396.


21. Han DC, Hoffman BB, Hong SW, Guo J, Ziyadeh FN. Therapy with antisense TGF-β1 oligodeoxynucleotides reduces kidney weight and matrix mRNAs in diabetic mice. Am J Physiol Renal Physiol. 2000. 278:F628–F634.
22. Gewirtz AM, Sokol DL, Ratajczak MZ. Nucleic acid therapeutics: state of the art and future prospects. Blood. 1998. 92:712–736.


23. Furdon PJ, Dominski Z, Kole R. RNase H cleavage of RNA hybridized to oligonucleotides containing methylphosphonate, phosphorothioate and phosphodiester bonds. Nucleic Acids Res. 1989. 17:9193–9204.


24. Beltinger C, Saragovi HU, Smith RM, LeSauteur L, Shah N, DeDionisio L, Christensen L, Raible A, Jarett L, Gewirtz AM. Binding, uptake, and intracellular trafficking of phosphorothioate-modified oligodeoxynucleotides. J Clin Invest. 1995. 95:1814–1823.


25. Agrawal S. Antisense oligonucleotides: towards clinical trials. Trends Biotechnol. 1996. 14:376–387.


26. Guvakova MA, Yakubov LA, Vlodavsky I, Tonkinson JL, Stein CA. Phosphorothioate oligodeoxynucleotides bind to basic fibroblast growth factor, inhibit its binding to cell surface receptors, and remove it from low affinity binding sites on extracellular matrix. J Biol Chem. 1995. 270:2620–2627.


27. Moon IJ, Choi KS, Choi YK, Kim JE, Lee YG, Schreiber AD, Park JG. Potent growth inhibition of leukemic cells by novel ribbon-type antisense oligonucleotides to c-myb1. J Biol Chem. 2000. 275:4647–4653.


28. Han SM, Kim EJ, Jeoung HS, Lee BY, Lee SS, Park KK, Kim HC. The effect of ribbon-type antisense oligodeoxynucleotides for transforming growth factor-β1 in unilateral ureteral obstruction. Korean J Pathol. 2002. 36:84–92.
29. Leehey DJ, Song RH, Alavi N, Singh AK. Decreased degradative enzymes in mesangial cells cultured in high glucose media. Diabetes. 1995. 44:929–935.


30. Kaname S, Uchida S, Ogata E, Kurokawa K. Autocrine secretion of transforming growth factor-β in cultured rat mesangial cells. Kidney Int. 1992. 42:1319–1327.


31. Akagi Y, Isaka Y, Arai M, Kaneko T, Takenaka M, Moriyama T, Kaneda Y, Ando A, Orita Y, Kamada T, Ueda N, Imai E. Inhibition of TGF-β1 expression by antisense oligonucleotides suppressed extracellular matrix accumulation in experimental glomerulonephritis. Kidney Int. 1996. 50:148–155.


32. Ying WZ, Sanders PW. Dietary salt modulates renal production of transforming growth factor-β in rats. Am J Physiol. 1998. 274:F635–F641.
33. Isaka Y, Tsujie M, Ando Y, Nakamura H, Kaneda Y, Imai E, Hori M. Transforming growth factor-β1 antisense oligodeoxynucleotides block interstitial fibrosis in unilateral ureteral obstruction. Kidney Int. 2000. 58:1885–1892.


34. Morishita R, Gibbons GH, Kaneda Y, Ogihara T, Dzau VJ. Systemic administration of HVJ viral coat-liposome complex containing human insulin vector decreases glucose level in diabetic mouse: a model of gene therapy. Biochem Biophys Res Commun. 2000. 273:666–674.


35. Agrawal S, Temsamani J, Tang JY. Pharmacokinetics, biodistribution, and stability of oligodeoxynucleotide phosphorothioates in mice. Proc Natl Acad Sci USA. 1991. 88:7595–7599.

